5 Unit 5
5.1 Water
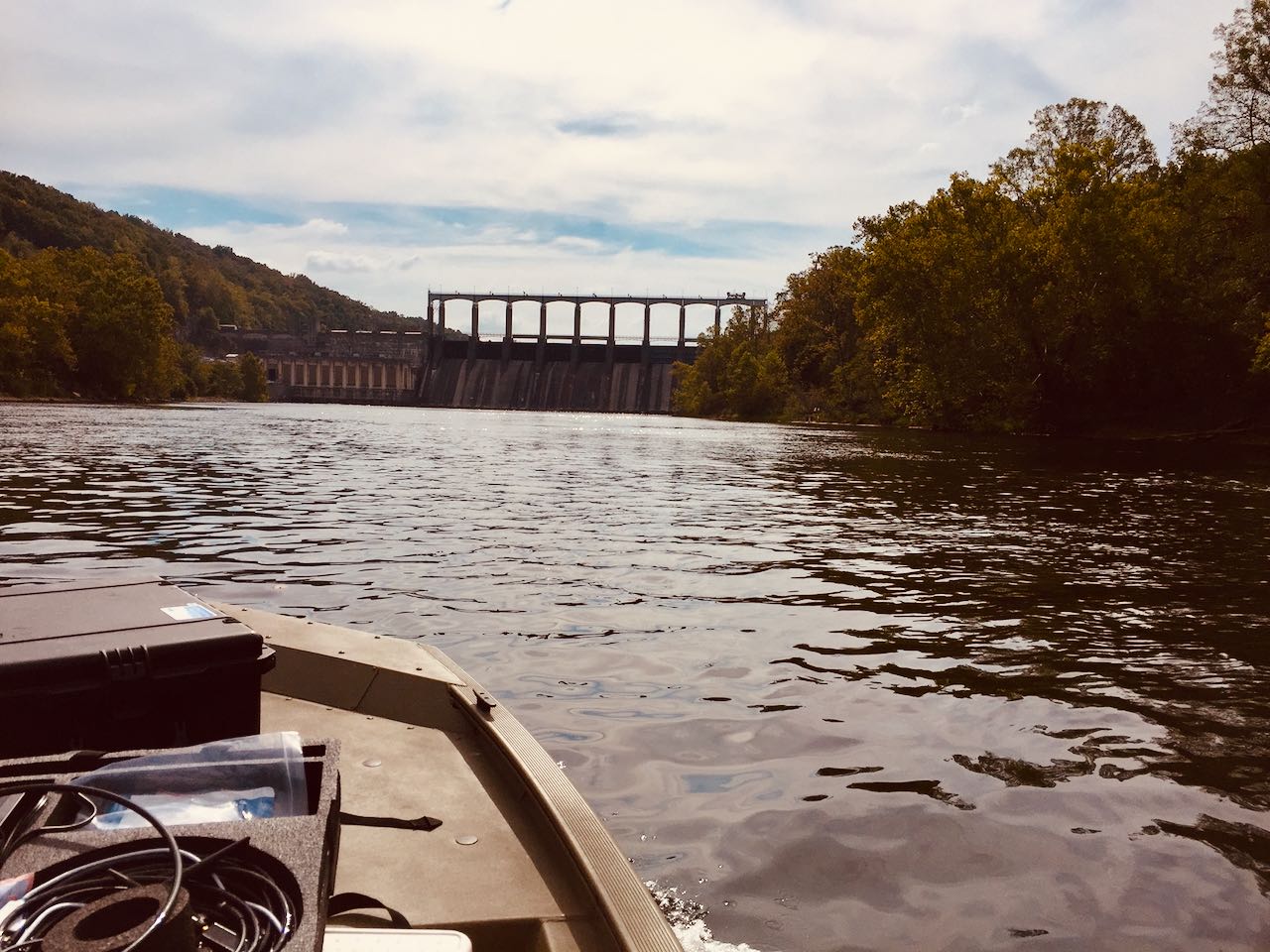
5.1.1 Background
In this chapter, we’ll introduce the basics behind water resources in our daily lives. We will discuss different sources and beneficial uses of water, the current and future challenges of meeting water demands, and how food, water, and energy relate to one another.
- What are the basic processes of the hydrologic cycle?
- How do scientists and engineers use the concept of a water budget?
- How and why have we altered the water cycle, and how are we addressing unintended consequences?
- What opportunities do engineers have to address water quantity and quality challenges?
- With water, food, and energy dependent on one another, what are sustainable approaches to meet competing demands?
Let’s first look at an interactive graph for streamflow below the Claytor Dam in the New River in Radford. Hydrologists, that is the scientists that explore the water cycle, measure the amount of water (volume, L3) moving through a stream or river over a given amount of time [T]. We use the term streamflow or discharge, which have fundamental units of L3/T. In the U.S., most hydrologists use units of ft3/s (abbreviated as CFS), whereas the units of m^3/s or L/s are used elsewhere (hint: when sharing a result, a best practice is to use a number between 0.1 and 1000 by using scientific notation). In the graph below, you can zoom into different times of the year and see when there was a lot of water moving through the New River, in contrast to drier periods. What are the primary controls on streamflow that result in variation over the year? In this module, we’ll explore these controls, and explore the role of water in Green Engineering.
The New River watershed is located in Southwest Virginia, with the headwaters (the smallest streams within the watershed) in North Carolina. Ultimately, the New River flows through West Virginia (a section of the river is a new National Park) and into the Ohio and Mississippi River before entering the Gulf of Mexico. The plot contains the daily average streamflow (that is, the volume of water per time) flowing near the city of Radford, Virginia below the Claytor Lake Reservoir. You can zoom in and see Hurricane Helene that came through in Fall of 2024, in contrast to the much lower summer-time streamflow.
5.1.1.1 Resources
USGS Water Science School - an online website that covers water basics. The USGS stands for the United States Geological Survey, an agency within the Department of Interior. The agencie’s vision is to “Lead the Nation in 21st-century integrated research, assessments, and prediction of natural resources and processes to meet society’s needs.”. In their science school, you’ll find curated background water information, access to data resources, and a range of case studies.
5.1.1.2 Primer: Definitions
- beneficial use = A legal term that varies by state, largely from pre-existing laws. In most states, these include drinking water, industry, irrigation, mining, hydroelectric, navigation, recreation, and wildlife. A detailed description from AICHE states that the term benificial use is “The cardinal principle of the (Prior) Appropriation Doctrine. A use of water that is, in general, productive of public benefit, and which promotes the peace, health, safety and welfare of the people of the State. A certificated water right is obtained by putting water to a beneficial use. The right may be lost if beneficial use is discontinued. A beneficial use of water is a use which is of benefit to the appropriator and to society as well. The term encompasses considerations of social and economic value and efficiency of use. In the past, most reasonably efficient uses of water for economic purposes have been considered beneficial. Usually, challenges have only been raised to wasteful use or use for some non-economic purpose, such as preserving instream values. Recent statutes in some states have expressly made the use of water for recreation, fish and wildlife purposes, or preservation of the environment a beneficial use.”
- evaporation = E [depth/time], phase-change of water from liquid to water vapor requiring solar energy. Thus, higher evaporation potential will co-occur in the same regions of the country where there’s high solar insolation see here.
- evapotranspiration = ET [depth/time], transfer of water through both evaporation and transpiration
- impervious = material that does not allowing water to move through it
- infiltration = I [depth/time], rate of water transfer from the land surface into the ground
- Julian day = An approach to plot daily values of long-time series between 0-365 days, where every Jan. 1st = 1, Jan. 2nd = 2, etc.
- Runoff = R [depth/time] the water during or after a precipitation event that does not infiltrate into the ground, but rather moves across the land surface to a body of water.
- streamflow or discharge = Q [L^3/T], measure of the volume of water moving past a location over a given amount of time (L3/T). Typical units are in ft3/s or mgal/d.
- surplus water = a term use in water balance models representing infiltration and runoff
- transpiration = water that moves from the leaf of the plant into the surrounding atmosphere
- U.S. Geological Survey = (e.g., USGS) Created by an act of Congress in 1879, the U.S. Geological Survey has evolved over the decades, matching its talent and knowledge to the progress of science and technology. The USGS is the sole science agency for the Department of the Interior. It is sought out by thousands of partners and customers for its natural science expertise and its vast earth and biological data holdings.
- water quantity = the amount of water available within a watershed for beneficial uses
- water quality = Water quality is a measure of the suitability of water for a particular use (e.g. drinking water, irrigation) based on selected physical, chemical, and biological characteristics. Just because streamwater looks clear and pristine does not mean that it’s safe to drink!
- watershed = (e.g., drainage area, L^2) the area of land that accumulates the excess rainfall and snowmelt (e.g., runoff) into streams and rivers. One way to think about this is if you are in a boat within any stream or river and look upstream to consider all of the land that contributes to the water flowing underneath you. Watersheds are typically determined by topography, where excess rainfall will flow downhill. [Note: watersheds are generally thought of from a surface-water perspective, and groundwater does not follow topographic controls.]
5.1.1.3 In the news
Water is the in the news all the time - from floods to droughts, poor water quality, to the importance of water, energy, and food in our daily lives. Below are some recent articles:
- SWIFT: Water Reuse in eastern Virginia, New York Times 20 October 2022
- Latest water research in ScienceDaily
- Importance of water modeling for proactive water resources management from Creamer Media’s Engineering News
- Example of a sustainable development built to ‘weather’ hurricanes. This story was written after Hurricane Ian caused significant loss of life and damage to Florida from NPR 6 October 2022
- Article on Groundwater Protection across the United States. Rules for groundwater management appear to be all over the place, and where they exist, big producers can get away with violating the law due to the low fines. Read more in “A Tangle of Rules to Protect America’s Water Is Falling Short” from the NYT
5.1.2 Major Sources - the Hydrologic Cycle
In elementary school, you were introduced to the hydrologic cycle. In a simplified version, the sun’s energy warms up water from lakes, streams, and the oceans, and when there’s enough energy, water evaporates into the air. Other sources of water vapor are from water leaving plants (transpiration), and evaporation from water within soil. As the water vapor rises into the atmosphere, the air cools and the water condenses into clouds, which release water as either rain, snow, or ice. When precipitation returns to the earth as rain, the water may infiltrate into the soil, or may stay on the ground surface and form a puddle or move across the ground surface (e.g., this is called runoff). In colder regions of the world, the majority of precipitation may fall as snow, where the resulting snow may be stored temporarily (e.g,, this is called the snowpack) until springtime air temperatures melt the snow. In polar regions, the snow can be stored long-term on glaciers and ice-fields.
Water that infiltrates from rain or melting snow can be used by plants, evaporated back to the atmosphere, or enter into groundwater. In turn, groundwater moves through rocks and soil and ultimately returns to a stream or river, providing an important source of streamflow, especially in the summer months. In fact, late August streamflow may be from precipitation from the previous winter or much longer ago! In snow-dominated regions, melting snow during the snowmelt period (April-July in the Northern Hemisphere) will often provide the majority of the yearly water to streams and rivers.
To illustrate this concept, let’s look at 3 different hydrographs. You might be asking what is a hydrograph - this is simply a 2-dimensional plot of time on the x-axis and streamflow (or discharge) on the y-axis, where discharge is in units of volume over time (here, cubic feet per second). The U.S. Geological Survey, funded through the department of Interior, operates over 8,500 sites across the U.S.. If you’ve taken a Water Resources class or are a kayaker, you may have come across the U.S.G.S. streamflow network. At each streamflow site, a sensor measures the stream’s water level at ~15-60 minute intervals, and the stream’s height is converted to streamflow (this is done be taking multiple hand-measurements of streamflow, and developing a mathematical regressional relationship between streamflow and water level). In the figure below, what do you notice about the shape and variabilty of the hydrographs?
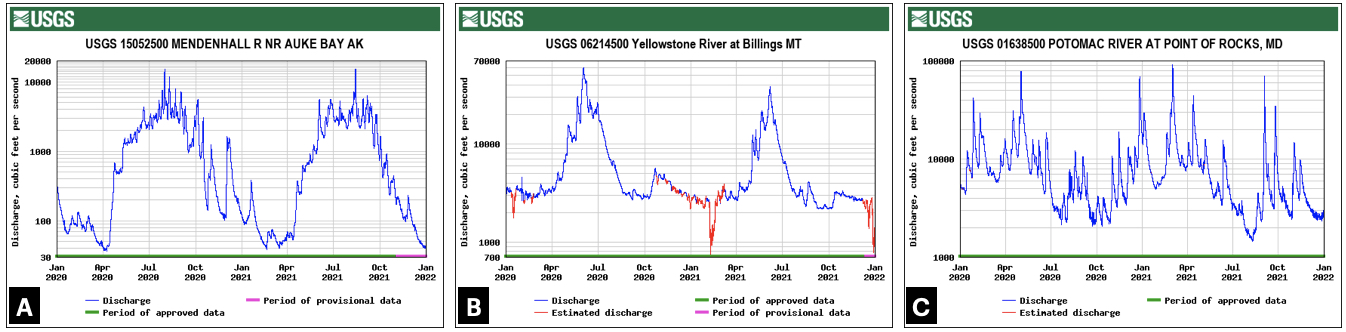
The Mendenhall River drains the Juneau Ice Field in Southeast Alaska, where the primary source of water is glacial melt. For the Yellowstone River, snowmelt and spring rain provides the majority of streamflow and similar to the Mendenhall there’s a distinct seasonal pattern. In contrast, the Potomac River’s streamflow is less variable, where rainfall is relatively consistent across the year. Evaporation and transpiration (known as evapotranspiration) will be highest in the summer period (July, August), resulting in lower streamflows. Storms and hurricanes will result in elevated streamflow, as seen in the early September 2021 rise in streamflow.
Runoff occurs when the rainfall rate (inches/hour) exceeds the infiltration capacity of the ground. For example, rain falling on a paved parking lot or street will simply not infiltrate into the ground below - the pavement is what we call ‘impervious’. What other surfaces where rain falls are impervious? In contrast, rain falling onto the forest floor, a dirt or grass road, or a field is more likely to infiltrate into the ground depending on factors including the infiltration capacity of the soil and the pre-existing soil conditions.
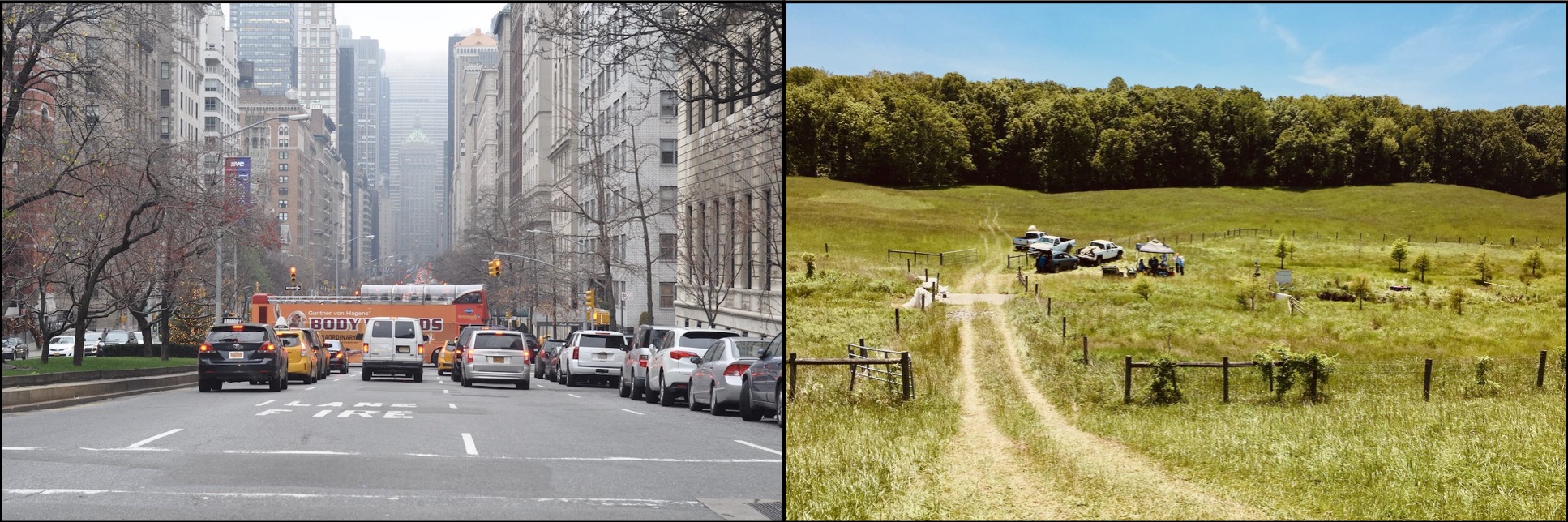
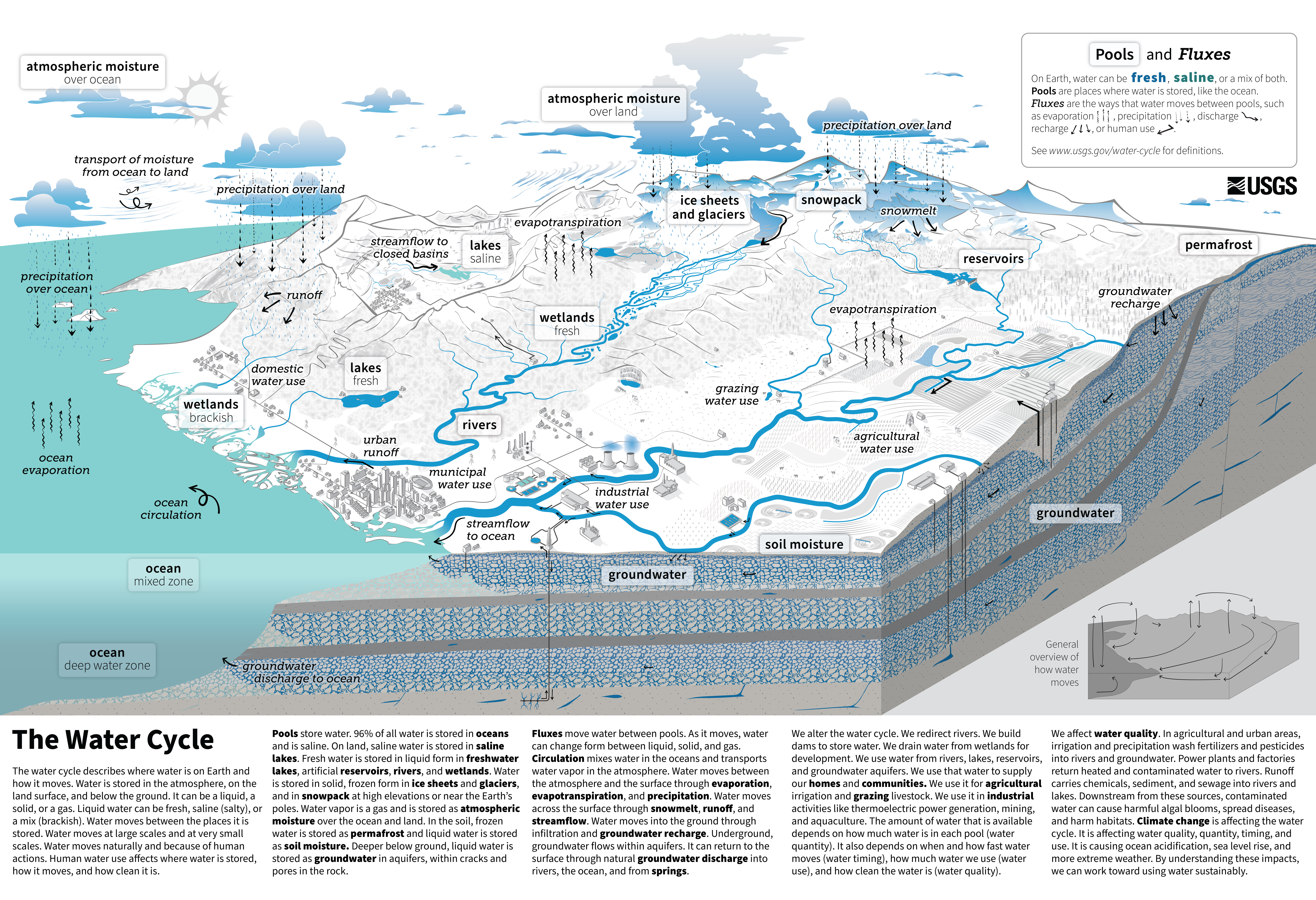
The following text describes the Water Cycle, including the terms pools and fluxes, and our collective impacts:
Pools store water. 96% of all water is stored in oceans and is saline. On land, saline water is stored in saline lakes. Fresh water is stored in liquid form in freshwater lakes, artificial reservoirs, rivers, and wetlands. Water is stored in solid, frozen form in ice sheets and glaciers, and in snowpack at high elevations or near the Earth’s poles. Water vapor is a gas and is stored as atmospheric moisture over the ocean and land. In the soil, frozen water is stored as permafrost and liquid water is stored as soil moisture. Deeper below ground, liquid water is stored as groundwater in aquifers, within cracks and pores in the rock.
Fluxes move water between pools. As it moves, water can change form between liquid, solid, and gas. Circulation mixes water in the oceans and transports water vapor in the atmosphere. Water moves between the atmosphere and the surface through evaporation, evapotranspiration, and precipitation. Water moves across the surface through snowmelt, runoff, and streamflow. Water moves into the ground through infiltration and groundwater recharge. Underground, groundwater flows within aquifers. It can return to the surface through natural groundwater discharge into rivers, the ocean, and from springs.
We alter the water cycle. We redirect rivers. We build dams to store water. We drain water from wetlands for development. We use water from rivers, lakes, reservoirs, and groundwater aquifers. We use that water to supply our homes and communities. We use it for agricultural irrigation and grazing livestock. We use it in industrial activities like thermoelectric power generation, mining, and aquaculture. The amount of water that is available depends on how much water is in each pool (water quantity). It also depends on when and how fast water moves (water timing), how much water we use (water use), and how clean the water is (water quality).
We affect water quality. In agricultural and urban areas, irrigation and precipitation wash fertilizers and pesticides into rivers and groundwater. Power plants and factories return heated and contaminated water to rivers. Runoff carries chemicals, sediment, and sewage into rivers and lakes. Downstream from these sources, contaminated water can cause harmful algal blooms, spread diseases, and harm habitats. Climate change is affecting the water cycle. It is affecting water quality, quantity, timing, and use. It is causing ocean acidification, sea level rise, and more extreme weather. By understanding these impacts, we can work toward using water sustainably.
–― U.S. Geological Survey Water Science School, https://www.usgs.gov/media/images/water-cycle-png
5.1.3 Water Budget
Let’s now consider how hydrologists put together a water budget. We’re going to use a simple water budget tool developed by J.P. Gannon and K. McGuire from Virginia Tech. In previous modules, we’ve examined powerplants and written mass-balance equations for inputs and outputs. Another example that Dr. Gannon uses that we can all relate to is a bank account. Try writing out an equation that accounts for the change in a bank account balance for a given month. What does it look like?
\[\Delta B = D - W\]
where B = balance, D = deposits, and W = withdrawals. We all know that our account balance varies over the year, where some months the balance goes up, and some months we spend more than we make and the balance goes down. Using the analogy, we can write a simplified water balance where we initially include just precipitation (P), evapotranspiration (PET), and soil storage (S):
\[\Delta S = P - ET\]
Here, this simple model is for a column of soil. Now let’s add some more terms, runoff (R) and infiltration (I):
\[\Delta S = P - ET-R-I\]
Hydrologists use methods to estimate evapotranspiration from meteorlogic (weather) data, including temperature, vapor pressure, and sunlit hours. Now that you have some background, let’s use a water balance model based on the above approach for changes in water storage available at a location. The model uses average monthly temperature and precipitation data, the soil water holding capacity, and the location of the site (estimates the number of sunlit hours for a month given the latitude, which in turn impacts the ET estimate). For this model, we’re going to lump R and I together, and call this surplus water.
\[\Delta S = P - ET-Surplus Water\]
In the model, we can adjust the soil water capacity (higher values will result in more storage), latitude (alters the monthly estimate of sunlit hours for the ET equation), temperature (higher temperatures, higher ET), and precipitation. Now that you have some background, let’s use a shinyapp created by Gannon and McGuire (Gannon 2021). Using your web-browser, navigate to the app and answer the following questions. The intent is to apply your basic understanding with real-monthly data to understand the seasonality of the water cycle, and how different places will have very different water balances based on their incoming precipitation and energy (affects the amount of ET).
Exercise
- Click on the Map tab, the blue dots there are all places you can run a water balance. The red dot is the current site location. Where is Burkes Garden, VA?
- What is the soil water holding capacity at Burkes Garden?
- What is the latitude of Burkes Garden?
- Go back to the Output Plots tab. How much water was input into the soil in January and February?
- Why is this? Reference the plots of total precipitation, temperature, and snow pack in your answer.
- What is the total amount of precipitation in March? How much water was input to the soil in March? What explains the difference?
- Switch the location of the budget to CAPE CHARLES 5 ENE VA. You can do this by starting to type the name in the site box, click the dropdown arrow and scroll to the site, or go to the map and click the location if you know where it is.
- Where is Cape Charles?
- Look at the storage plot for Cape Charles. What is the value of soil storage from Jan-May? What parameter does this represent? How do you know?
- Look at the surplus water line in the Outputs plot and compare it to the soil storage line in the Storage plot. When the soil storage line is flat, what can you say about the surplus water line? Why do you think the values behave this way?
- Keep looking at the surplus water line and the soil storage line. What happens to the surplus water line in the outputs plot when the soil storage line in the storage plot dips? Why do you think this is?
- Look at the precipitation plot. What is the wettest month of the year in Cape Charles? Is that also the month with the most surplus water in the outputs plot? Why?
- Which month has the most surplus water? Why?
- Look at the Evapotranspiration (ET) line in the outputs plot and the Temperature plot. Explain the relationship between the two lines. Why are the two lines related in this way?
- Based on what you’ve learned on the seasonality of water stored in the soil, imagine you’re advising on a consulting project for a small farm. What considerations related to water would you bring to the conversation, and why?
- Use your knowledge of the water balance and the answers above to help you fill in the blanks in the following 2 paragraphs:
When precipitation falls it can either fall as _____ or _____. If the temperature is sufficiently cold, the precipitation falls as _____ and becomes _____ in the model. If the temperature is warm enough, the precipitation falls as ______ and goes into _____ storage. Additionally, when it gets warm, water stored as _____ will melt and go into _____ storage.
Water can leave _____storage either through _____ or _____. If _____ storage is below the available water holding capacity of the soil, then water only leaves through _____. If _____ storage reaches the available water holding capacity of the soil, it can leave through _____ or _____. If it leaves by _____ it can either recharge groundwater or go to a stream or river.
Other hydro apps/sites of interest
5.1.4 Human modifications to Stream and Rivers
Water diversion is not a new concept, and it’s not something that’s relegated to dry regions (e.g., Western U.S.). Humans have been diverting water long distances for a long time. For example, the Romans built a series of aqueducts over 2,000 years ago. Today, large dam construction is largely outside the U.S. (e.g., Amazon River Basin, Mekong River Basin). For example, the Three Gorges Dam was build in 2008, with a surface area of 419 square miles and 22,500 MW generating capacity. While the dam is a large source of hydropower production, the construction and operation of the dam also comes with negative impacts. Given the demand for hydropower production, future efforts are underway to balance the benefits and costs, including the ecological impacts (Fu et al. 2010).
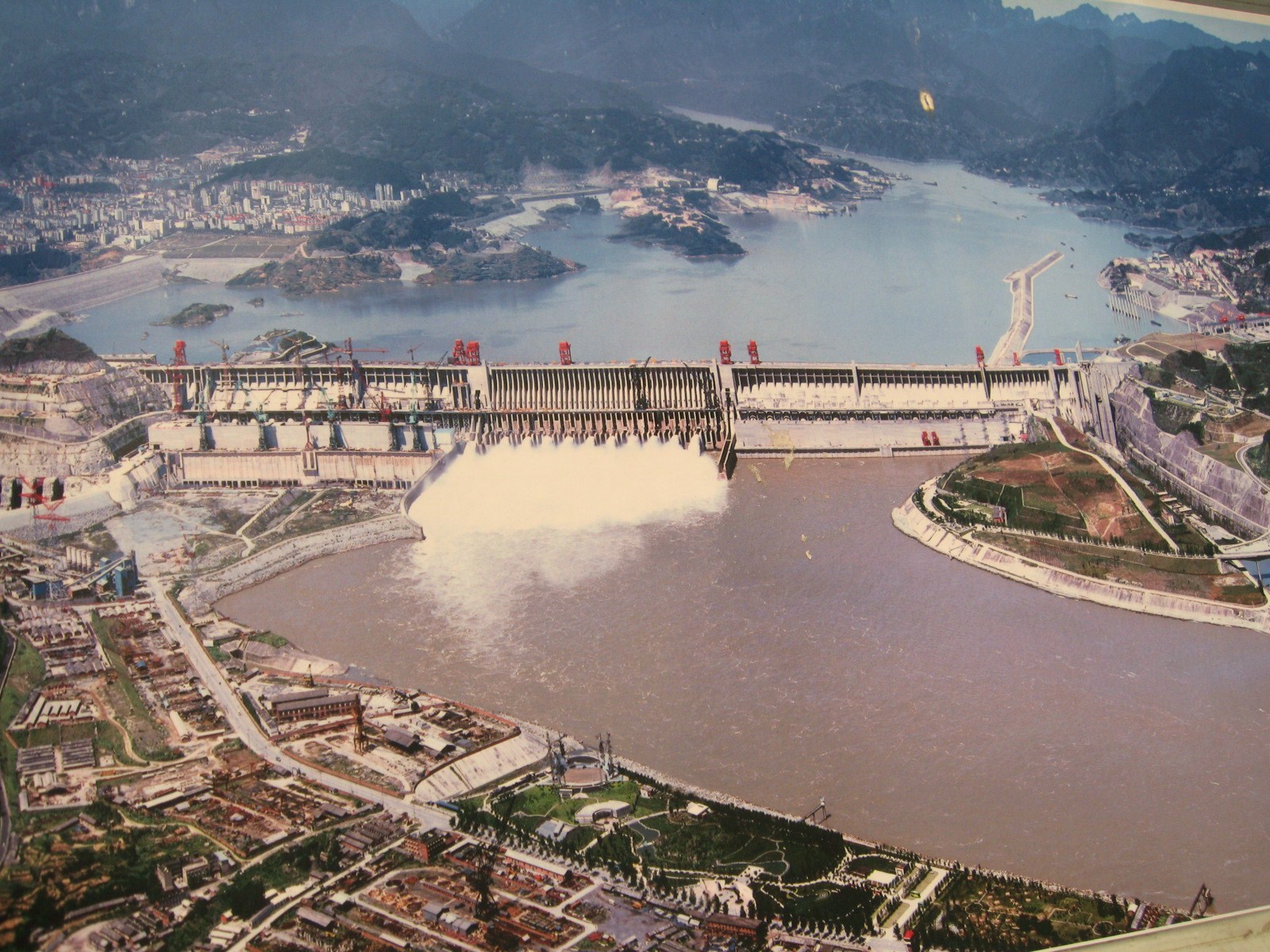
https://www.flickr.com/photos/pvcg/3412711352
In the U.S., we often hear of the large infrastructure projects from the 1940s and 1950’s. For example, the Colorado River was ‘harnessed’ through a series of canals and dams to provide water and electricity to California and Arizona. A quote from the 1993 book Cadillac Desert describes the flow of water to supply cities and agriculture:
“In the West, it is said, water flows uphill toward money. And it literally does, as it leaps three thousand feet across the Tehachapi Mountains in gigantic siphons to slake the thirst of Los Angeles, as it is shoved a thousand feet out of Colorado River canyons to water Phoenix and Palm Springs and the irrigated lands around them.”
–― Marc Reisner, Cadillac Desert: The American West and Its Disappearing Water
While we may think of large projects being contained to the western U.S., have you ever wondered how major cities in the Eastern U.S. provide drinking water? For example, let’s consider New York City. The current population of New York City is approximately 8.4 million people. We can look up data for the total withdrawals of freshwater for the State of New York in Table 5 of Dieter (2018):
New York total population served by public water, 2015: 17,300,000
Total Freshwater Withdrawls: 2,420 Mgal/day
Using this data, we can calculate the average water requirements (e.g., domestic use) per person in New York, and then estimate the total water requirements for NYC in both Mgal/day and ft3/s (CFS). By converting to CFS, you can get an idea of just how much water is needed.
Variable | Units | Value |
---|---|---|
NY State population | million (M) | 17.3 |
NYC population | million | 8.4 |
NY State water withdrawals | Mgal/day | 2420 |
Domestic Water Use, average state | gal/person | 140 |
NYC domestic water requirements | Mgal/day | 1175 |
NYC domestic water requirements | CFS | 1818 |
Because the Hudson River is tidal (that is, a mixture of fresh and salt water) well above NYC, NYC obtains it’s freshwater source from far upstream. Take a look at the image below that highlights the watersheds supplying NYC. What do you notice?
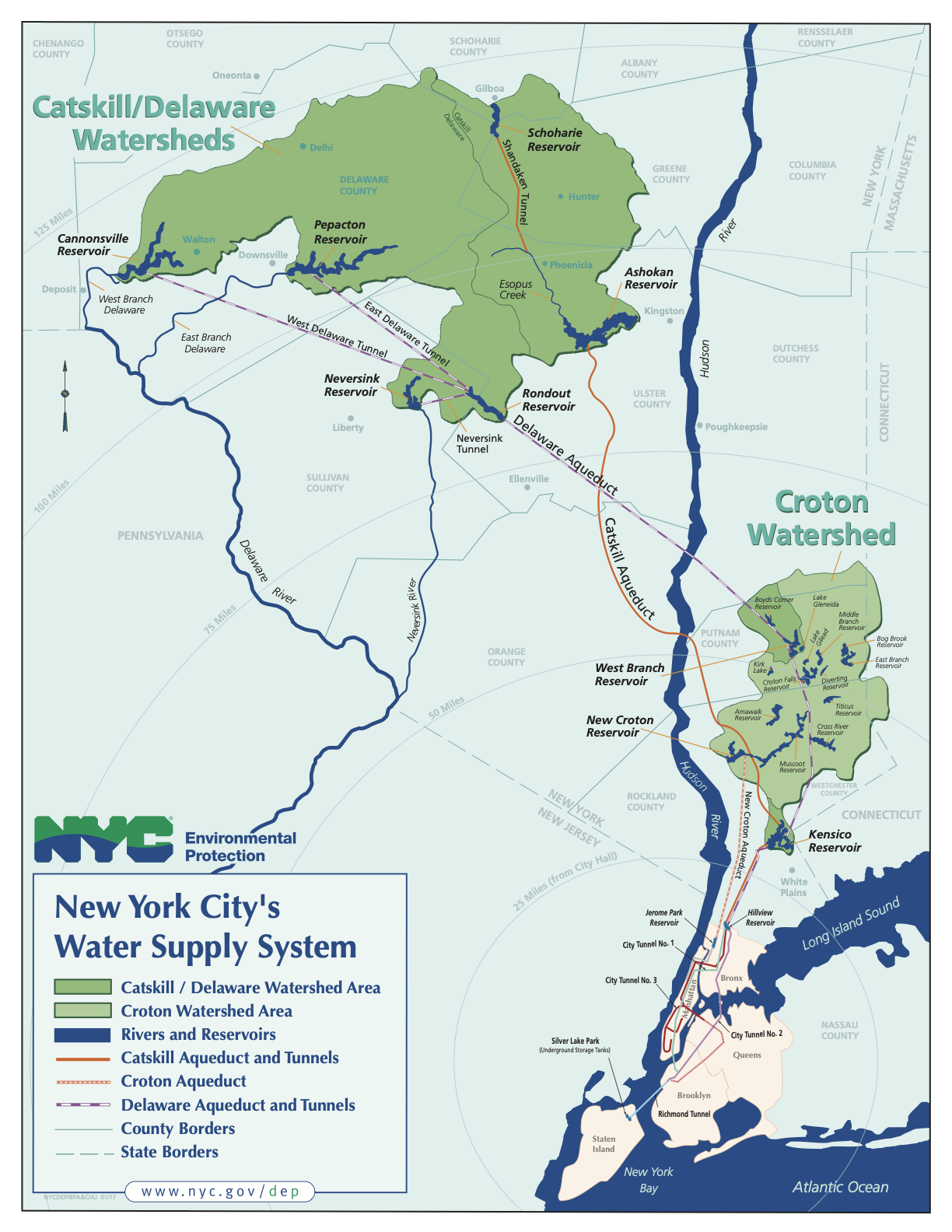
I’m guessing you see that water is piped through a series of tunnels and stored in reservoirs from quite a long distance from NYC. For example, the Delaware Aqueduct was completed in 1944 and has a capacity of ~500 Mgal/day. Water is diverted from the Delaware River Watershed and flows via gravity to a series of reservoirs in the Croton Watershed. These large water diversion projects were attractive to supplying NYC with water because of how ‘clean’ the water was in these watersheds - that is, there was good water quality. Given the cost of water treatment, protecting the water supply in these distant watersheds from pollution is more cost-effective than building a large plant. Since the 1990’s, NYC has spend over $1billion dollars (Hu 2018) to protect the water supply, including an active watershed protection program, from improvements to household septic systems to large scale municipal wastewater treatment plant upgrades. From a sustainability lens, this large water project is diverting approximately 8 of the mean Delaware’s mean annual streamflow. What are the things you like, and the things you wonder about, from a triple bottom line to consider?
I like …
- that the upstream watershed protections are keeping the water clean, benefiting the local people and in-stream beneficial uses
- that NYC is using old, existing infrastructure to save taxpayer money and minimize the need to build a large, new plant that would require materials and long-term energy use
- …
I wonder…
- how local farmers and landowners landuse is impacted, and if it reduced their net incomes
- how the water diversions during low summer flows impact the local streams and rivers other beneficial uses
- …
5.1.4.1 Regulations and approaches to dam impacts on streamflow
Today, there are over 80,000 dams in the U.S. alone. As described above, dams provide multiple benefits for society. However, with most things in life, there are also negative consequences. For example, imagine if you relied on fishing to feed and support your livelihood. During a fish’s lifetime, their are life stages of growth that rely on specific habitat types and streamflow to support a healthy fish population for any given species. While engineers from the 1920’s and 1930’s understood that dams would alter streamflow, there was not as much of a recognition of these other impacts. It wasn’t until 1968 that the Wild and Scenic Rivers Act was passed, which kept certain rivers free-flowing (e.g., dam free). If you navigate to the Wild and Scenic Rivers web portal, you can look up where these protected rivers are. In 1986, the U.S. Congress passed the Electric Consumer Protection Act, which states the following during recertification of hydroelectric facilities:
“Directs the Commission, when issuing such licenses, to give equal consideration to: (1) energy conservation; (2) fish and wildlife protection; (3) recreational opportunities; and (4) environmental quality. Requires that licenses be granted upon the condition that the project adopted shall, in the judgment of the Commission, be the one best adapted to a comprehensive plan which encompasses: (1) fish and wildlife protection (including spawning grounds and habitat); and (2) irrigation, flood control and water supply. Requires the Commission to consider when making such judgment: (1) the extent to which a project is consistent with a comprehensive plan for waterways; (2) the recommendations of governmental agencies; (3) the recommendations of Indian tribes affected by the project; and (4) the electricity consumption efficiency improvement program of specified applicants. Requires the Commission to solicit recommendations from such agencies and Indian tribes upon the receipt of a license application.”
In the early 1990’s, researchers began to examine how we’ve altered the nation’s streamflow (e.g., Poff et al. (1997)). Let’s look at a specific example of streamflow variation over the year pre-and post-dam construction on the Roanoke River.
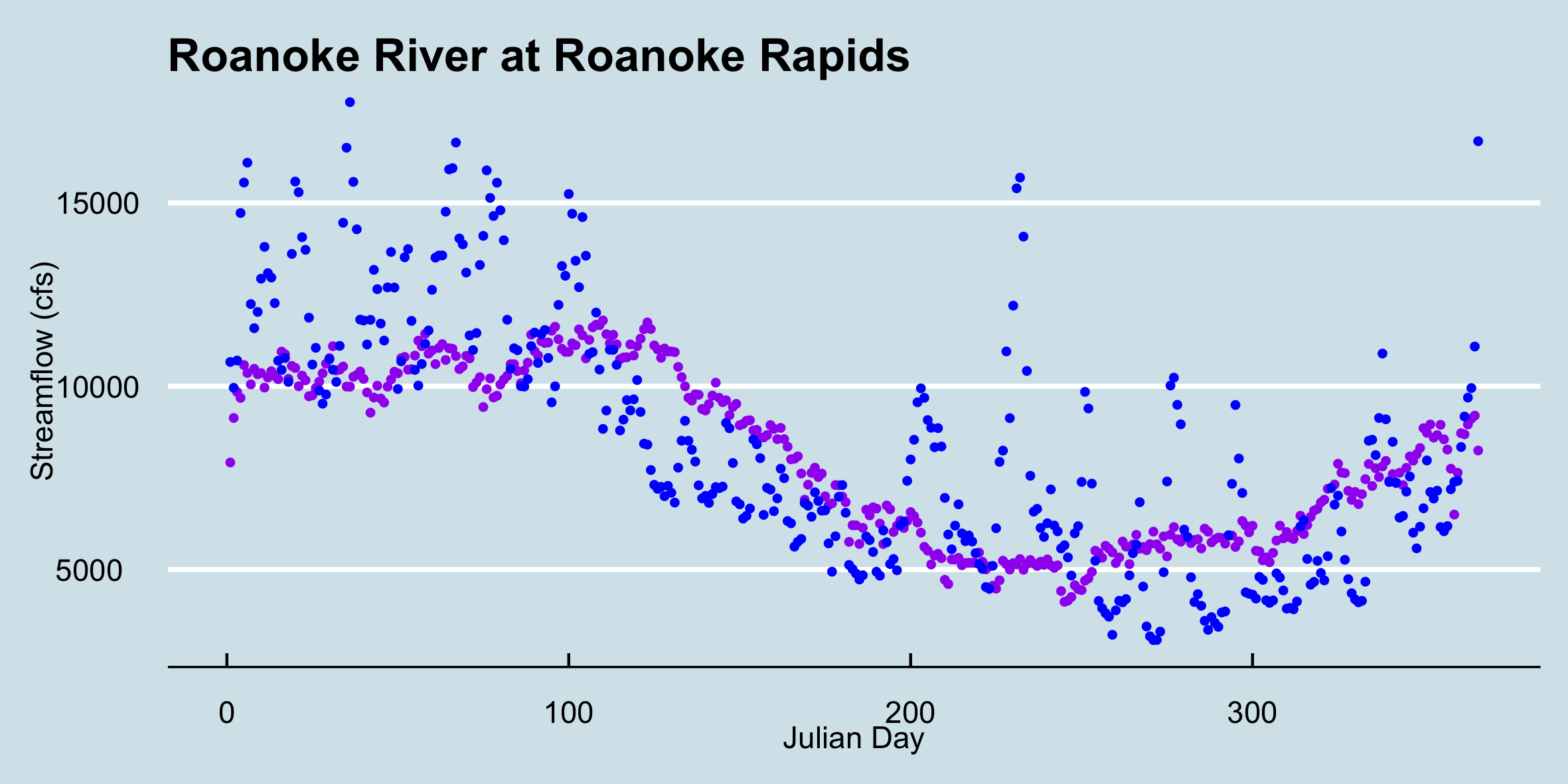
Exercise
- Which series of of points are pre-dam and post-dam?
- How have higher flow periods changed in the winter months? How might the water stored over the winter be used today?
- What might the higher summer streamflows post-dam impact in-stream habitat?
- At ~ day 100 (April), daily streamflow begins to decrease. Why is this happening (e.g. specific hydrologic process)?
- How might the delay in stream recession (decrease in April) post-dam impact in-stream habitat/biota? If more water was released post-dam in April to mimic pre-dam conditions, what are the implications for hydropower production?
We’ll look at some solutions to hydrologic flow modifications later in this unit that are beginning to positively impact our freshwater resources, while balancing the other benefits including hydropower.
5.1.4.2 Current Challenges
Society’s 2 largest challenges in water resources relate to amount (quantity) and composition (quality). Let’s briefly introduce these concepts. First, let’s start with the terminology:
Amount (Water Quantity - is there enough for the given use and others?)
Composition (Water Quality - is it safe for the intended purpose?)
5.1.4.3 Amount (Water Quantity - is there enough for the given use and others?)
Let’s begin with water quantity. We all need a minimal amount of water to survive on a daily basis. Health guidance suggests we need between 3-4 liters of water per person daily just for drinking; this doesn’t include the water we use for other aspects of our life, from toilet flushing to showering. Let’s do a thought exercise for each of our own average water use: Estimate your daily water needs in gallons/day. Include toilet use, showers, dishwashing, drinking, and 1 load of laundry per week. As you estimate this, consider the following: - Where does the water come from? - How does it get to where you use it, and is there energy required in this process? - How much does the water weigh? - What many 1-liter nalgene bottles would your daily water need require?
Variable | Units | Value |
---|---|---|
Toilet flushes | times per day | 5 |
Toilet flushes | gallons per flush | 1.6 |
Flow Rate Shower | gallons/minute | 2.5 |
Shower Length | minutes | 5 |
Shower Length | minutes | 5 |
Dish washing flow rate | gallons/minute | 2 |
Dish washing Length | minutes | 10 |
Laundry | gallons/load | 20 |
Number of loads per week | number/week | 1 |
Water footprint | gal/day | 44 |
Water footprint | lbs | 367 |
# Nalgenes | nalgene bottle/day | 167 |
That’s a lot of water, and it weighs a lot. How does your use compare to this estimate? Now imagine you were tasked with collecting water for a family of 4 on a daily basis. What would you change? For us, with easy access to water, assume you are going on a camping trip and need to bring water for the trip.
Beyond our domestic water supply, water quantity is also critically important for other beneficial uses. For example, farmers require water during the growing season (May - September) for food production and summer energy peaks. Summer periods also tend to have the lowest streamflows (see above), thus water availability is stretched the thinnest during the summer months, especially during droughts when demand increases (e.g., higher irrigation needs). The estimate above is of your direct water use = that is, water that we see flowing through the taps. What about the embedded water in the material (e.g., cotton) and energy we use? Or the food we eat?
Water Project provides a water footprint calculator that’s easy to use. Here are some estimates of common foods:
Product | Embedded water, gallons |
---|---|
lettuce, 4 oz. | 7 |
avocado, 4 oz. | 35 |
quinoa, 4 oz. | 135 |
coffee, 1 cup | 66 |
pork, 4 oz. | 188 |
beef, 4 oz. | 463 |
The embedded water in the products we use and processes we rely on is critical to water sustainability across the world - and is frankly important for national security. But let’s illustrate this using a simple example that may resonate with some of us - guacamole. Who doesn’t love fresh guacamole? When I go to the grocery store, it’s a sad day when there are no avacados available. Over the last 2-decades, avacados have grown in popularity for the average U.S. consumer, resulting in greater avacado trade from 0.4 Mt in 2000 to almost 2.0 Mt in 2016 Caro et al., 2020. Ås described by Caro et al., water used to grow avacados resulted in the virtual water flow (or embedded water) from regions with low water availablity (Mexico, Peru, Chile). While avacado production provides local economic stability, the concern becomes if acacado production is using water faster than it’s being replenished within the local watersheds. What happens if water is not available for drinking water? Or for other beneficial uses? This example highlights the interconnectedness of our global society, and how our own purchasing decisions at the grocery store rely on water availablity far from where we live.
In our daily lives, water use is not something most of us think about and take for granted that our tapwater is safe. In 2020, there were approximately 2 billion people worldwide that do not have access to safe drinking water, which represents 1 in 4 people worldwide. While the population has increased from 2000 - 2020 by 26.2%, the percentage of people without access to safe drinking water has decreased -13%. Thus, there’s been a modest increase over time, but there’s still significant improvements to be made. [See section on SDGs and improvement]
5.1.4.4 Composition (Water Quality - is it safe for the intended purpose?)
Water quality, or simply the composition of what’s in water, is just as important as water quantity. We long ago realized that contaminated water results in poor-human health outcomes, from the short-lived stomach flu to arsenic toxicity in drinking water prevelant in Bangeldesh. For drinking water in the U.S., our water needs to be within a safe pH range, free from organic contaminants, and below accepted levels for trace metals and bacteria. We are continually learning about new constituents in our water, from PCPs (personal care products) such as antibiotics to caffeine. After we flush water down the drain, wastewater treatment processes remove solids, and clean up water to reduce nutrient concentrations before discharging back into a stream. For irrigation water, the salt content is measured to maintain crop production and soil health. For streams and rivers, excess nutrients and higher temperatures can result in local eutrophication (excess algal growth), which reduces dissolved oxygen and impacts the health of fish and other aquatic species.
What controls water quality? Here, you can think through the sources and reactions that contribute to water’s composition. First, let’s imagine water melting from a glacier in Southeast Alaska. The composition of this meltwater is impacted not only by what’s living in or on top of the glacier (yes, there are microbes on the ice), but also the underlying bedrock below the ice (that is, the geology) and any atmospheric deposition from the air. Over the last decade, mercury and organic carbon in glacial melt water has been directly linked to coal combustion as far away as Asia (Stubbins et al. (2012)). While we may think of Alaskan Glaciers as untouched, they are not.
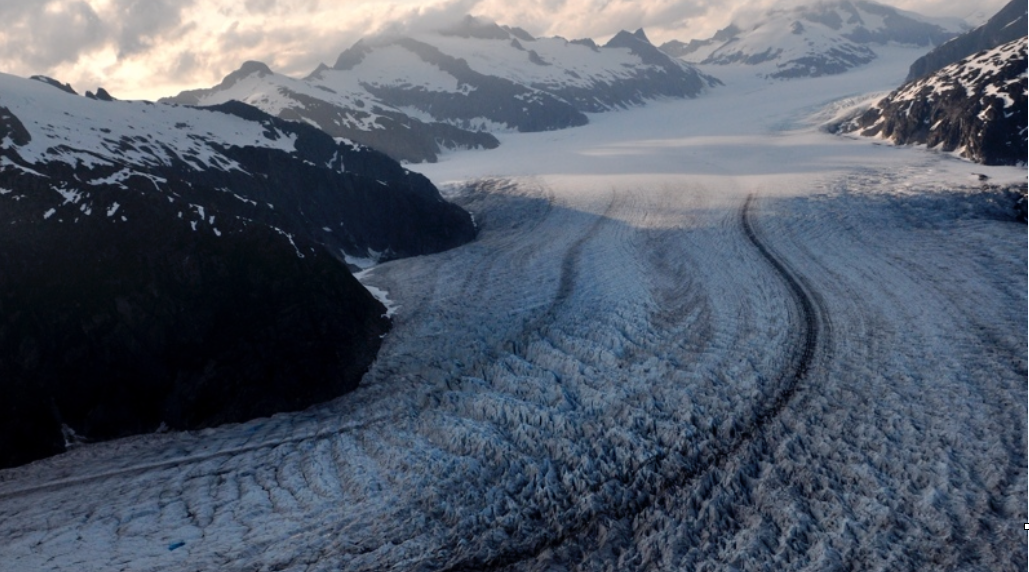
Within watersheds, surface runoff can mobilize material on the ground, from dirt during a construction project to fertilizers on a field to fallen leaves within a forest. Water the infiltrates into the ground will bring dissolved material into the ground, where there’s a potential for both some removal via filtration to mobilization of sorbed solutes (here, think of what would happen if you add water to a cup of soil, shake, and then filter - the water would likely have a brown-yellow color from the mobilization of organic matter in the soil). Increasing nutrient removal is one approach to improving water quality. Infiltrated water also picks up material through rock dissolution, and thus the underlying geology is another source that contributes to water composition. Direct discharges from facilities into streams and rivers are regulated in the U.S. (see section on Clean Water Act) through the NPDES National Point Discharge Elimination System. You can think of a point source as a pipe that directly enters a stream - these can be from treated waste water effluent to industrial wastewater. Non-point sources are from diffuse sources, as surface runoff is transported over the landscape. Examples include the mobilization of nutrients, sediment, and animal waste.
Note: This section is incomplete at this time.
5.1.4.5 Beneficial Use
Virginia has a legal description for the beneficial uses of water - “Beneficial use” means both in-stream and offstream uses. In-stream beneficial uses include, but are not limited to, the protection of fish and wildlife habitat, maintenance of waste assimilation, recreation, navigation, and cultural and aesthetic values. Offstream beneficial uses include, but are not limited to, domestic (including public water supply), agricultural, electric power generation, and commercial and industrial uses. A protected beneficial use will require an amount of water (quantity) that has a particular composition (quality).
5.1.5 Legal protections
In the U.S., we benefit from the Clean Water Act. The Clean Water Act expanded in 1972 and addressed point-source pollution explicitly through permitting processes (NPDES, see above), and recognized the need for addressing non-point source pollution. What galvanized congress to act? Imagery and news stories of rivers that were literally on fire (Cayuga River Fire) and an oil spill off the coast of California spurred the Environmental Movement, using images of wildlife covered in oil and the Cayuga River to bring attention to the American Public. Three years later, congress passed the 1972 Clean Water Act with the aim of: - eliminate pollutant discharge into freshwater (e.g. streams, rivers, lakes) - achieve water quality levels that are both swimmable and fishable Significant investments were made in our nation’s Wastewater Treatment Facilities to eliminate the discharge of pollutants into freshwaters, and to achieve water quality levels that are fishable and swimmable. This landmark legislation has improved human well-being across the U.S. and is a testament to collective action. While CWA has been successful, addressing non-point pollution has not been nearly as successful (without a pipe entering a stream, it’s more difficult to pinpoint the ‘sources’).
5.1.6 SDGs
Beyond the U.S., the United Nations adopted the Sustainablity Development Goals in 2015. These goals include the following:
- 6.1 By 2030, achieve universal and equitable access to safe and affordable drinking water for all
- 6.2 By 2030, achieve access to adequate and equitable sanitation and hygiene for all and end open defecation, paying special attention to the needs of women and girls and those in vulnerable situations
- 6.3 By 2030, improve water quality by reducing pollution, eliminating dumping and minimizing release of hazardous chemicals and materials, halving the proportion of untreated wastewater and substantially increasing recycling and safe reuse globally
- 6.4 By 2030, substantially increase water-use efficiency across all sectors and ensure sustainable withdrawals and supply of freshwater to address water scarcity and substantially reduce the number of people suffering from water scarcity
- 6.5 By 2030, implement integrated water resources management at all levels, including through transboundary cooperation as appropriate
- 6.6 By 2020, protect and restore water-related ecosystems, including mountains, forests, wetlands, rivers, aquifers and lakes
- 6.a By 2030, expand international cooperation and capacity-building support to developing countries in water- and sanitation-related activities and programmes, including water harvesting, desalination, water efficiency, wastewater treatment, recycling and reuse technologies
- 6.b Support and strengthen the participation of local communities in improving water and sanitation management
The infographic below illustrates the current state of SDG 6 in 2022.
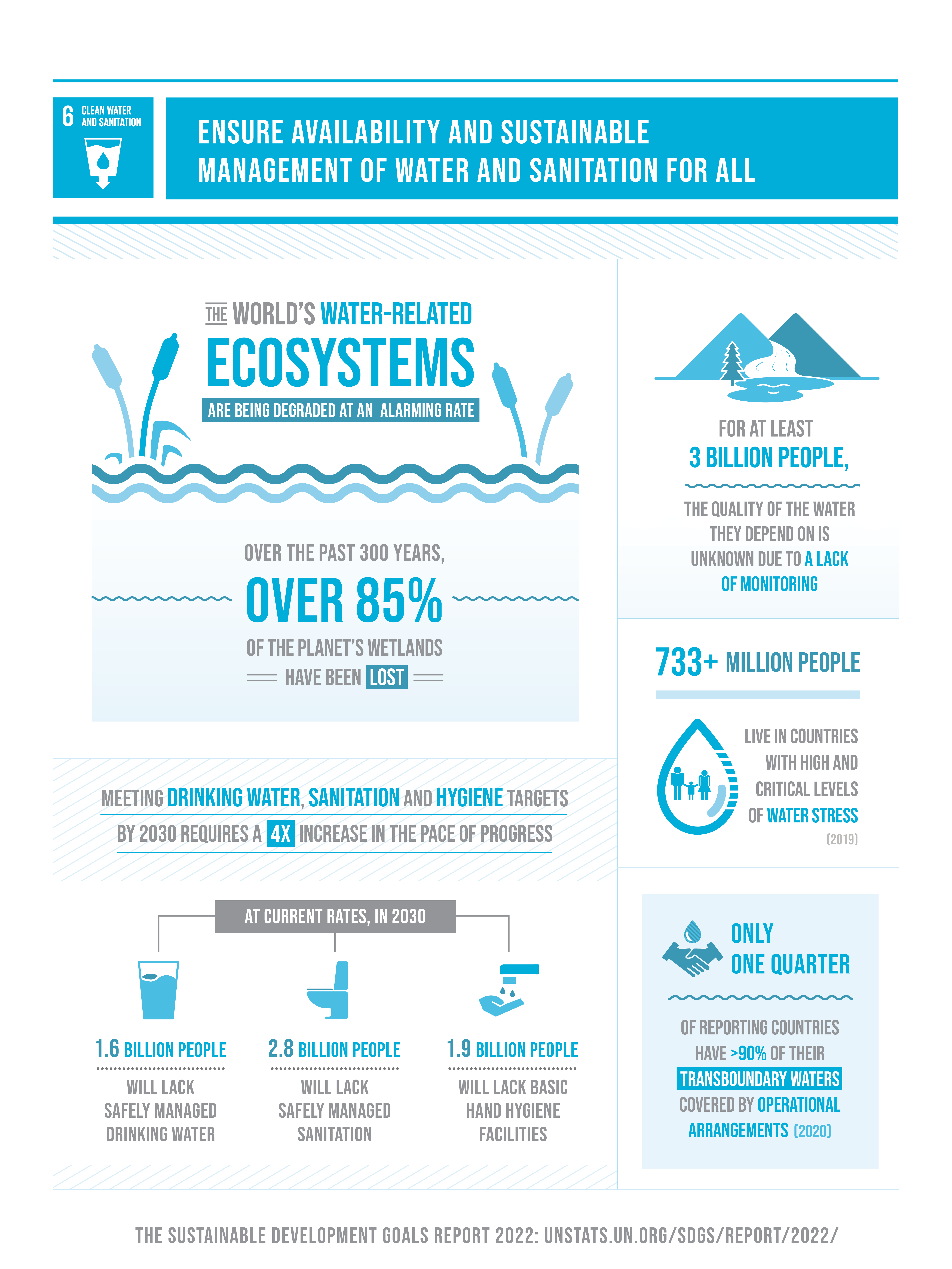
The Sustainable Development Goal 6 goes beyond drinking water, sanitation and hygiene to also address the quality and sustainability of water resources, which are critical to the survival of people and the planet. The 2030 Agenda recognizes the centrality of water resources to sustainable development and the vital role that improved drinking water, sanitation and hygiene play in progress in other areas, including health, education and poverty reduction. Below is a graphic that illustrates countries without access to clean water.
5.1.7 Solutions
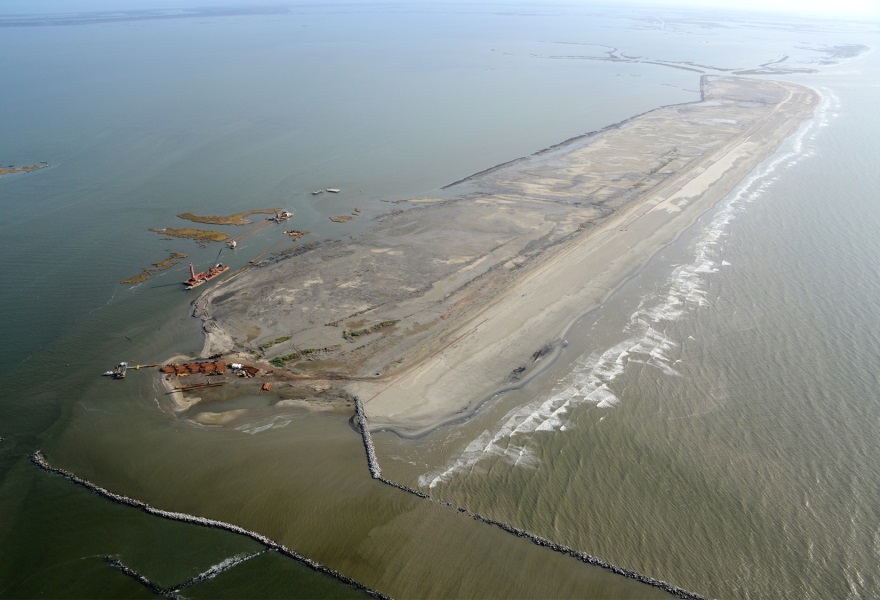
Nature based solutions leverage ecosystem services to slow water down and clean water up. Our largest challenges related to water are too much, too little, or too dirty for the stated beneficial use. Green infrastructure also leverages ecosystem processes, but generally requires more engineering. Why are these approaches gaining traction across the world? Largely because as climate variability increases resulting in more floods and droughts, hard infrastructure can only reduce downstream flooding so much. For example, over the last century one, engineers have build levees to protect adjacent farmland and cities from flooding. These levees work well when they work - and if they are high enough. However, when failure occurs, failure is catastrophic leading to loss of human life, devastation of communities, and severe economic consequences. The story below illustrates just how fast waters can rise and the resulting impacts.
5.1.7.0.1 Flash Flooding in Kentucky - what are the impacts?
One example is in Troublesome Creek located in Knott county in Kentucky. In late July 2022, several inches of rain fell on top of saturated soil, quickly resulting in rapid surface runoff to the adjacent streams and rivers. The resulting floods in the region were devastating to the local communities, where a local river North Fork Kentucky River at Whitesburg increased over 14 feet in 7 hours! One of the businesses that was destroyed by the flooding was Troublesome Creek Guitars, a company whose mission is to bring jobs to folks that are recovering from drugs and alcohol. Patagonia released an impactful short video that highlights some of the human hardships in coal regions with a feature on Troublesome Creek.
5.1.7.0.2 Solutions
Below are a brief summary of nature based solutions (NBS) and other engineering solutions to address water-related issues. This recent book (Cohen-Shacham et al. (2016)) provides more examples and case studies for NBS that address food and water security, human health, reduce hurricane risks, and enhance economies and human well being, and this resource from the NRDC provides additional examples and stories. Most of these either slow water down, bring contaminants into contact with bio(reactive) surfaces, or reduce contaminant sources (e.g., smart farming). Below are of some of the common approaches used today:
Protection of water sources. Much of our drinking water supplies come from watersheds that are close to drainage divides. Strategies to limit agricultural and urban runoff into these freshwater lakes and streams prior to extraction for a beneficial use are employed across the world. The New York Case study above is one example. Check out the story of Bill Cooper, a farmer in Wythe County, Virginia.
Reduce erosion and increase infiltration in agricultural landscapes. Traditional farming practices often uses tilling at the end of the growing season to prepare for the next season. No-till practices have gained popularity in the farming community, which improves soil water holding capacity (reduce risk to droughts), decrease fertilizer use (reduce expenditures), and increase productivity. Just ask Terry McAlister, farmer in North Texas: >“My goal is to improve my soil so I can grow a better crop so I can make more money,” said Terry McAlister, who farms 6,000 acres of drought-stricken cropland in North Texas. “If I can help the environment in the process, fine, but that’s not my goal.” > > –― Terry McAlister, N.Y. Times March 9, 2015
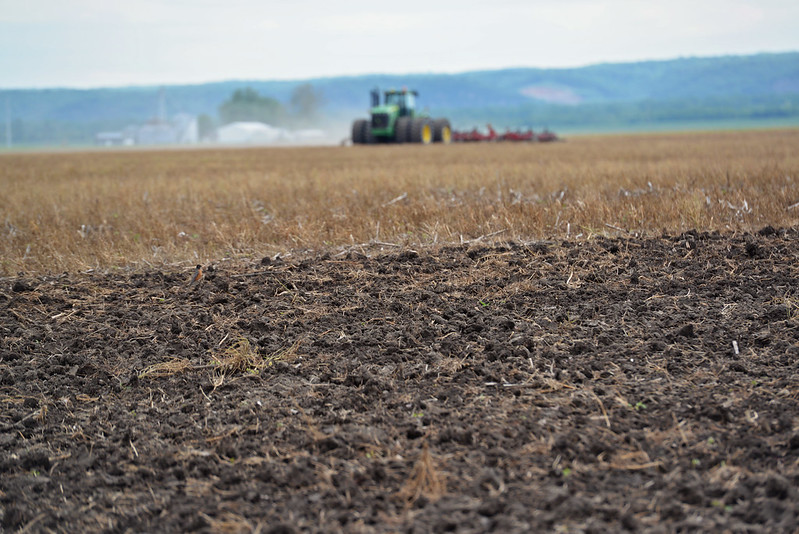
Increase riparian buffers. CREP is a federal program that provides financial incentives to farmers to enhance riparian buffers, that is the land adjacent to a stream or river. By keeping animals out and increasing vegetation, erosion is reduced and some nutrients are filtered prior to entering the stream. To find out more, navigate to the CREP page or find information relevant to your state (e.g., Virginia). This extension publication describes how stream buffers work: largely by slowing water down!
Edge-of-Field management. Approaches use to collect and filter runoff from farms before entering a nearby stream. This extension publication provides information on using bioreactors that increase microbial removal of nitrogen.
Stream and Floodplain Restoration. Many Biological Systems Engineers provide design and guidance during stream and floodplain restoration. Stream restoration can be designed to improve local habitat (e.g., for fish), decrease bank erosion, and improve the stream for recreation and human well-being. Engineering approaches include cutting back the banks and bank stabilization, to creating pools and steps within a degraded stream. Tree planting is also generally done simultaneously to increase habitat, provide shade for the stream (and reduce temperatures), and increase infiltration. In floodplain restoration, the goal is often 2-fold: to decrease downstream flood peaks by storing water during floods, and to increase biological removal and settling by bringing flood water to biologically active soils.
Case study: Stroubles Creek is a small stream that begins in the hills above Blacksburg, Virginia, flows through and under downtown Blacksburg (as in right under the Lyric movie theater, Gillies), under the drill-field, and emerges above the Duck Pond. The stream then flows under Highway 460 and by Foxridge through Virginia-Tech Foundation land towards the New River. The stream was listed as impaired for aquatic life in 1995, and Dr. Cully Hession and Dr. Thompson persued funding to restore the section of stream adjacent to Foxridge see video here on the StreamLab project. Prior to 2010, cattle had direct access to the stream, streambanks were eroding, and the stream was both warm and lacked biodiversity. The pictures below of before and after are remarkable in terms of the impact of the tree-plantings, and the stream is showing signs of recovery. This aerial imagery during a storm illustrate how flood-water moves on and off the floodplain, providing opportunities for water quality improvements.
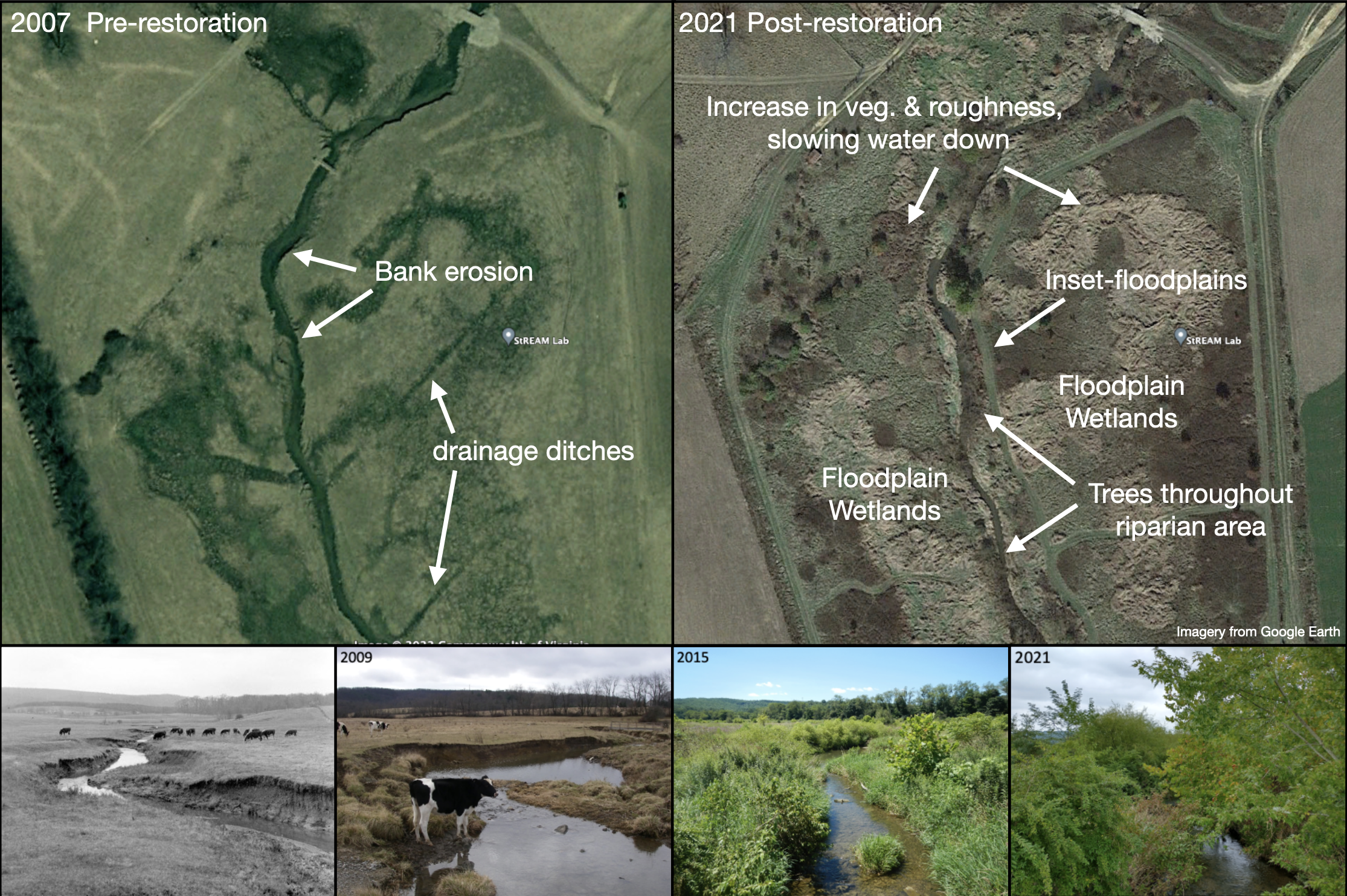
Increasing infiltration : From permeable pavers to bioretention. Thse engineering approaches require more engineering, but can serve to both reduce peak flow from floods (that is river levels) and clean up water through filtration and biological removal.
Water harvesting / gutter disconnection. These approaches have been around since the dawn of time. Water harvesting is done in many regions of the world to collect and store water at the household/farm scale (although in the Western U.S., rainwater harvesting is illegal - you’d be using someone’s water allocation!). Gutter disconnections and temporarily storing water in rain barrels is used to slow water down.
Example: Consider a house that is a 2,500 square foot house (or 35’ x 35’). For a 1” rain, how many water barrels would you need? What barriers exist to limit effectiveness?
Answer: You’d need 16 water barrels, assuming each held 50 gallons. Barriers include aesthetics and draining after rains.
Wetland protection and restoration. Wetlands are unique habitats that provide many imporant ecosystem services that we rely on: water storage, nutrient removal, long-term carbon storage, and wildlife habitat (e.g. waterfowl). Federal wetlands protection works towards a no net wetland loss in size or function. While it’s pretty simple to measure the size of wetland loss that needs to be replaced, replacing the same size wetland with no net loss in function (ecology, function, structure) in a place that limits loss to cultural ecosystem services is challenging. The EPA has outlined a list of best practices for wetland restoration - you’ll see the list is long with many that are not easy to measure.
Urban water management. Urban areas are challenged with runoff due to the high impervious surfaces from parking lots, driveways, and buildings. Stormwater detention ponds and infiltration trenches are examples employed to reduce the harmful impacts of runoff. Other examples include installing underground pipes to store water on site (see below) or building ‘soft’ conveyance features in contrast to traditional flood control concrete lined channels. This extension publication provides an overview of stormwater management.
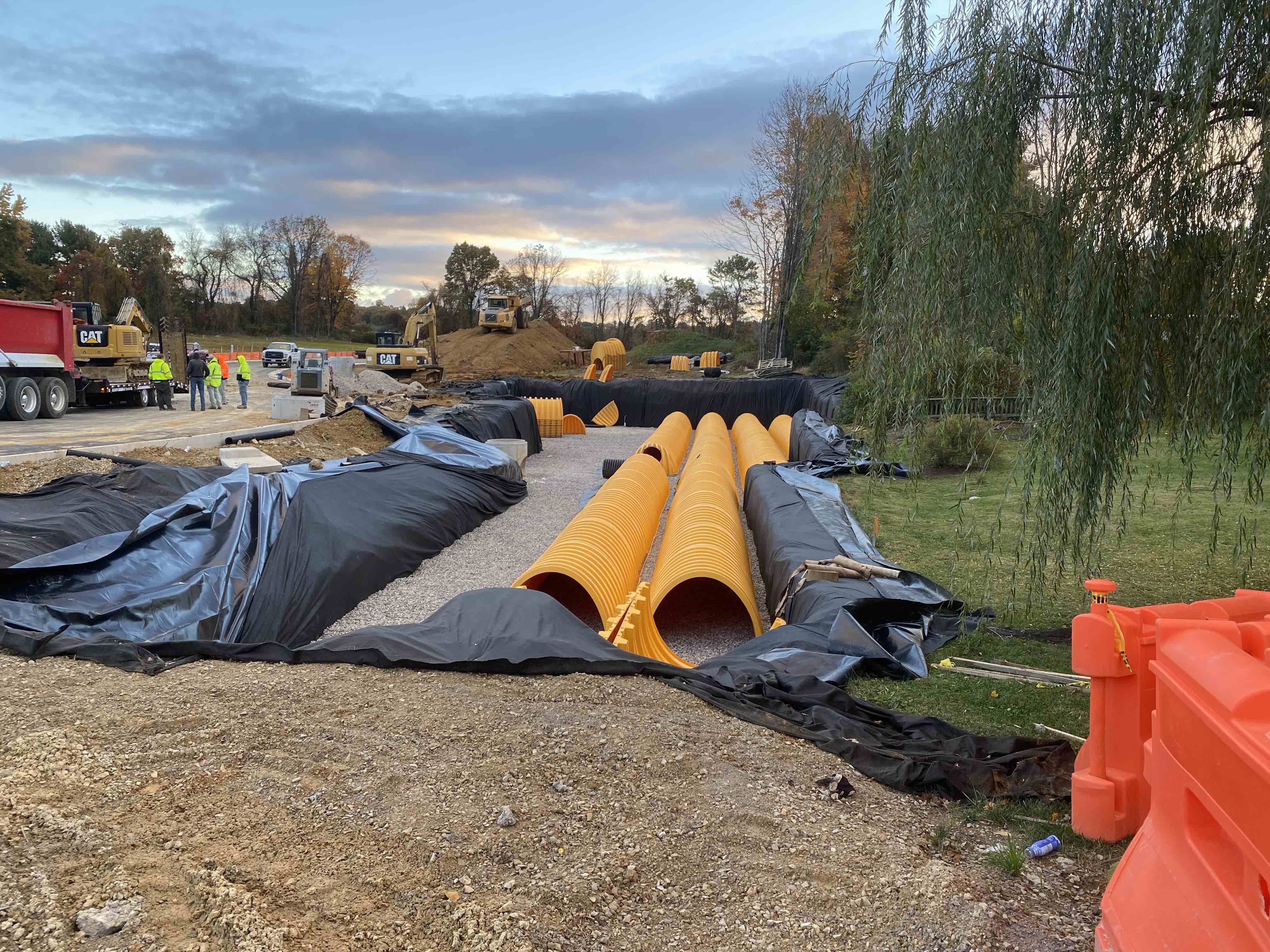
Smart Farming. Where are fertilizers needed to support growth? When is the optimal time to irrigate based on soil moisture? What areas of a field don’t make sense to grow crops? These are all questions that “sensors”smart” sensors are now used for, from soil-moisture sensors in irrigation management to drones and remote sensing. Virginia Tech is leading smart farming in the mid-Atlantic to improve farmer’s livelihoods and improve our environment, providing solutions that become a win-win.
Water Conservation approaches. From low-flow toilets to more efficient irrigation, these methods reduce the amount of water needed across major sectors. Less water used during our daily lives not only reduces water extraction, but also saves energy by reducing pumping and filtration needs. Water reuse systems are also being developed and applied in many sectors, especially in water-scare regions. For example, the city of San Diego treats wastewater for non-potable uses (irrigation, industrial uses).
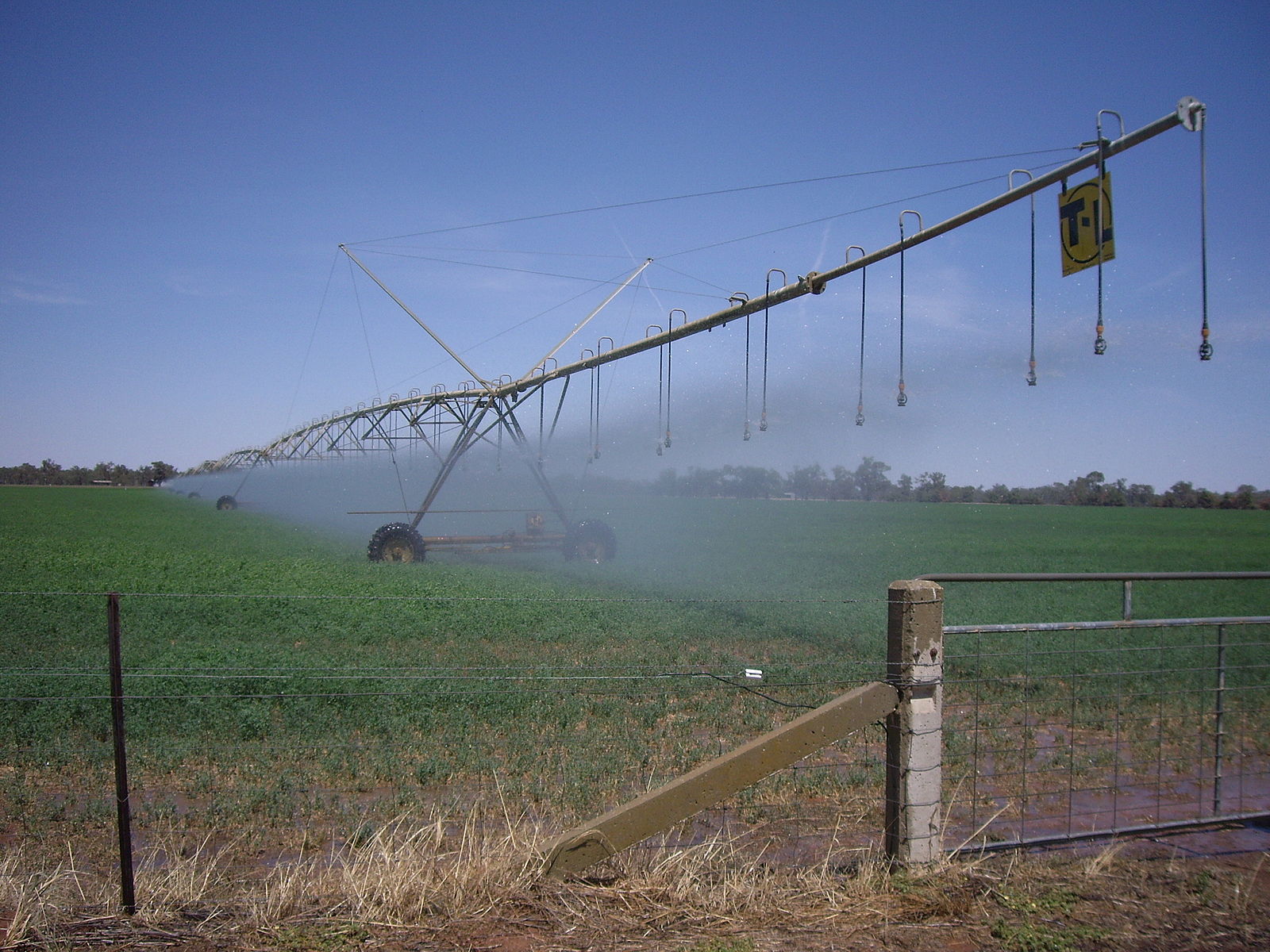
5.1.8 Water-Energy-Food Nexus
Water, food, and energy. How are they related? - Water (plant growth) & energy (pump water, produce fertilizer, run equipment) are required for food production - Water is used to grow plants for biofuels and in thermo-electric & hydroelectric power plants - Energy is required to pump and filter water for public-water supply and other uses
In meeting the SDGs as the world’s population grows to above 10 billion people, the United Nations and ASABE are promoting a combination of sustainable practices, renewable energy, and integrated water management.
[ADD here]
5.1.9 Data Sources
- Streamflow & Water Quality Network U.S. Geological Survey
- Current Precipitation & Weather National Weather Service
- Streamflow forecasts National Weather Service
- Snow Coverage NOAA
- Historic Weather PRISM, Oregon State
- Drought Monitor USDA and UNL
- Flood History CU-Boulder INSTAAR
- Landcover and Landuse in Chesapeake Bay CB Conservancy, U.S.G.S., U. Vermont
5.2 Food Systems
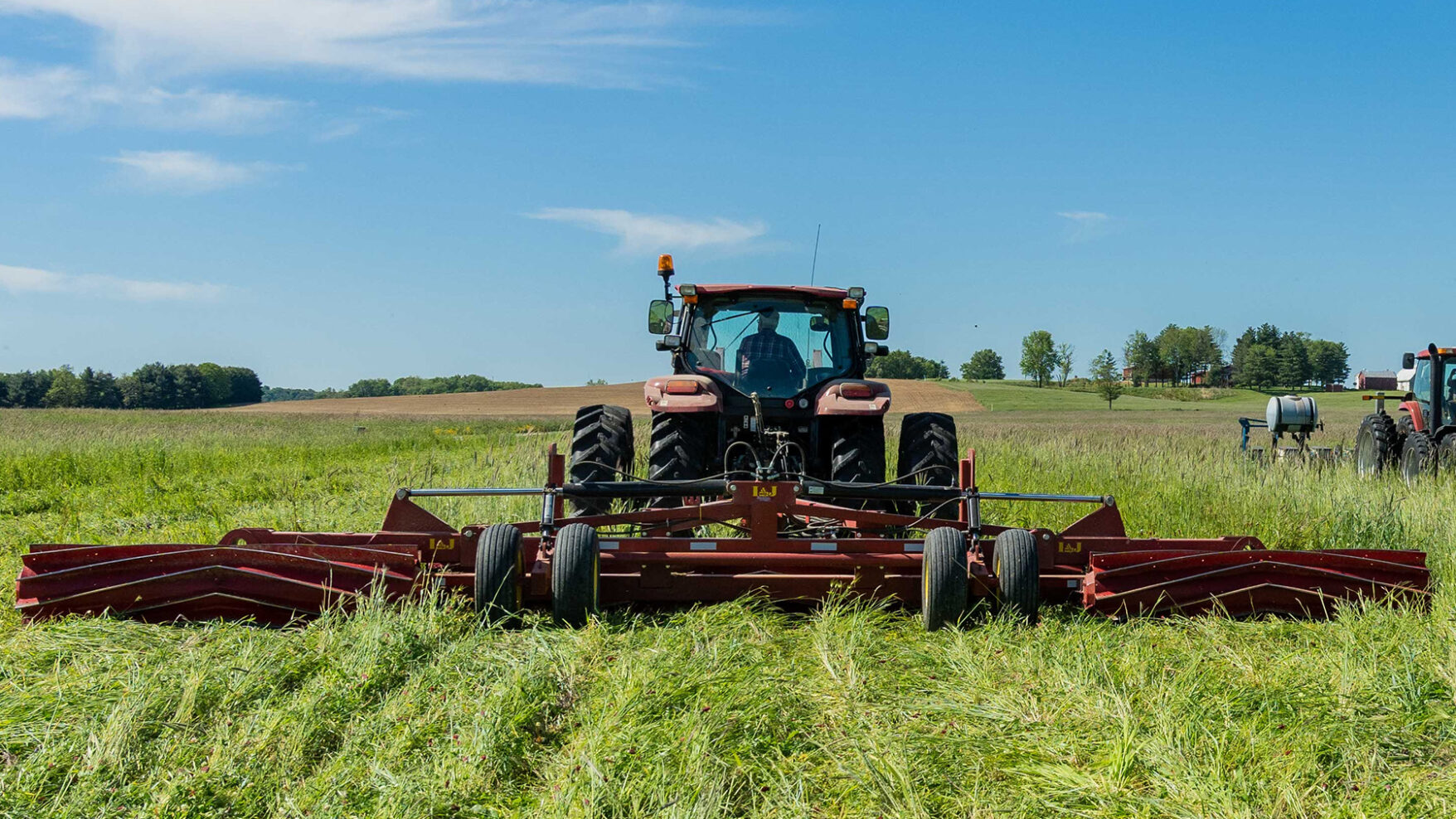
Key Concepts
-
Soil quality
-
Food waste
-
Nexus between food, water, and energy
Key Questions
-
How much food do we need for the future?
-
What are the tradeoffs for food production and the environment?
-
How can technology improve food and agriculture?
-
What are issues and options for food waste?
5.2.0.2 Primer: Definitions
https://www.usda.gov/oce/sustainability/definitions
- food system = Agriculture and food systems are the intact or whole unit made up of interrelated components of people, behaviors, relationships, and material goods that interact in the production, processing, packaging, transporting, trade, marketing, consumption, and use of food, feed, and fiber through aquaculture, farming, wild fisheries, forestry, and pastoralism. The food and agriculture system operates within and is influenced by social, political, economic, and environmental contexts. - As defined by the U.S. in its Global Food Security Strategy
5.2.0.3 In the news
- A fascinating story by ProPublica into 20 farmers that use a lot of water; one farm uses more water than Las Vegas. And the catch is that the water isn’t used to grow food for direct human consumption.
Podcasts
Good Food - from food culture to the people who bring us food
Risky or Not - is a particular food safe to eat?
5.2.1 Background
In this module, we’ll introduce the basics behind food systems.
We’ve watched and learned many things over this semester. As you all finish this semester, I wanted to come back to the premise of hope. What brings us hope is you all - we believe you all have the capacity to make a difference in the complex world we live in. Some of you may wonder where you will end up, even be worried about this. In this video, the farmer Jeannie Blasberg provides some salient advice: “It’s going to be a long and winding journey, but you will arrive at the place you are meant to be”
Like water and energy, it’s easy to take food for granted, but we know that an adequate supply of nutritious food is not available or affordable for everyone around the world. In fact, the United Nations Food and Agriculture Organization, FAO estimates that approximately 2.3 billion people in the world were moderately or severely food insecure in 2021 while over 10% percent of the global population faced food insecurity at severe levels. Moreover, data suggests that progress in recent years had stalled for Sustainable Development Goal (SDG) 2 – Zero Hunger which targets to end hunger, achieve food security, improve nutrition and promote sustainable agriculture around the world.
According to the World Resource Institute, WRI, see Figure 5.1, there is a significant shortfall between the amount of food we produce today and the amount needed to feed everyone in 2050. Populations models indicated that there will be nearly 10 billion people on Earth by 2050, an increase of approximately 3 billion more people than there were in 2010. With rising incomes across the world, history suggests that people will consume more resource-intensive, animal-based foods. At the same time, we urgently need to cut greenhouse gas (GHG) emissions from agricultural production and stop conversion of remaining forests to agricultural land. Sustainable food production, by 2050, will require three gaps to be close:
A 56% food gap between crop calories produced in 2010 and those needed in 2050 under “business as usual” growth scenario
A 600 million-hectare land gap, an area nearly twice the size of India, between global agricultural land area in 2010 and expected agricultural expansion by 2050
An 11-gigaton GHG mitigation gap between the modeled agricultural emissions in 2050 and the target level needed to hold global warming below 2°C (3.6°F), the level considered necessary for preventing the worst climate impacts.
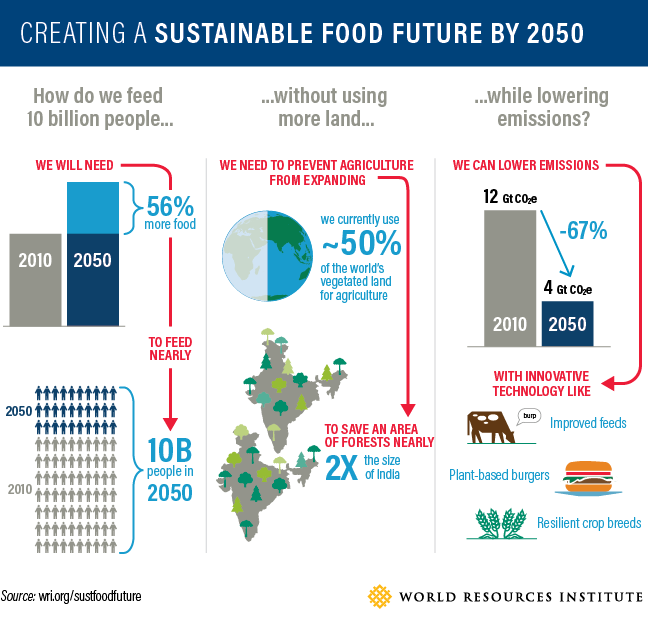
Figure 5.1: Sustainable Food Challenges. Source: World Resources Institute (WRI) - https://www.wri.org/insights/how-sustainably-feed-10-billion-people-2050-21-charts
There are no simple or easier answers to close the food, land and GHG mitigation gaps. WRI research on how to create a sustainable food future has also identified 22 solutions that need to be simultaneously applied to close these gaps. The relative importance of each solution varies from country to country. The solutions are organized into the following 5 categories:
Reduce growth in demand for food and other agricultural products
Increase food production without expanding agricultural land
Protect and restore natural ecosystems
Increase fish supply
Reduce GHG emissions from agricultural production.
The challenge of feeding 10 billion people sustainably by 2050 is much harder than people realize. All 22 solutions need to be implemented to close the food, land and GHG mitigation gaps. In addition to meeting these needs, there are additional triple bottom line (TBL)co-benefits for farmers, society and human health. The Creating A Sustainable Food Future report details these solutions and the associated GHG emissions reductions shown in Figure 5.2 . Like previous module topics, it’s important to realize that many of these solutions are not solved by engineering and technology, but rather by other political, social, economic, and cultural behaviors. We will focus on engineering solutions while recognizing the limitations and trade-offs of technology.
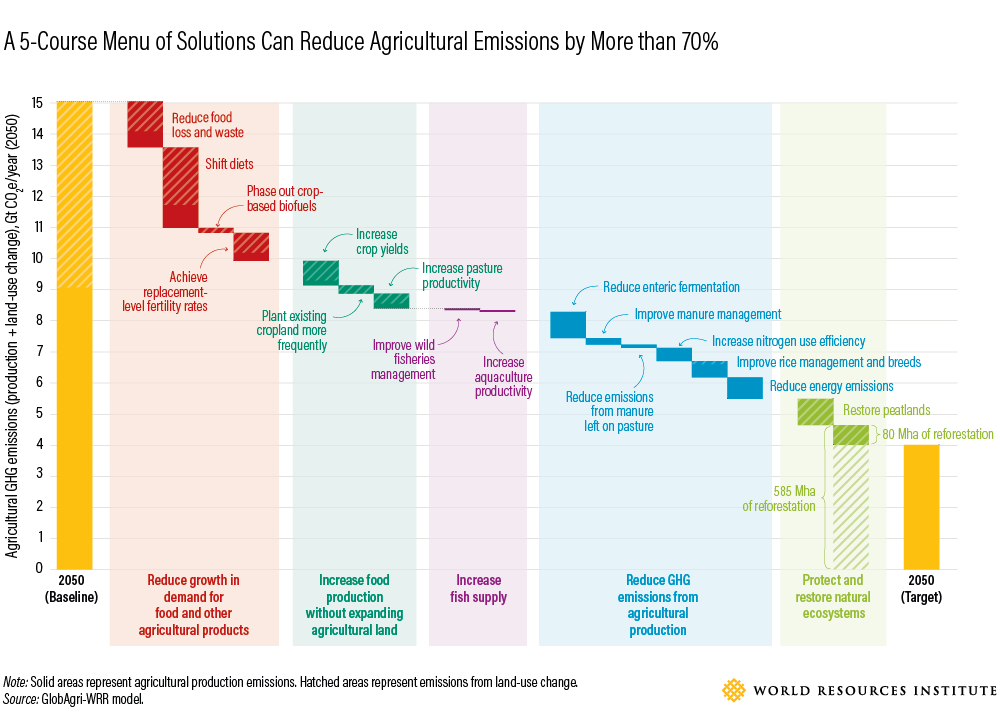
Figure 5.2: Solutions to Reduce Agricultural Emissions . Source: World Resources Institute (WRI) - https://www.wri.org/insights/how-sustainably-feed-10-billion-people-2050-21-charts
Food systems are complex with many different components across the life cycle. Like energy and water, many aspects of the food life cycle are hidden from plain site of citizens since they happen upstream or downstream of the use phase. The life cycle stage for food production can be broken down into the following stages: * Extraction (Farming, Hunting, Fishing, Gathering)
Manufacturing
Packaging
Transportation
Use
Disposal
Typically, agriculture encompasses the first stage of extraction including the growth, harvest, and initial processing of food products. While we typically think of farms, these systems are much more diverse and can include orchards, oceans, forests, greenhouses, and many other types.
As we discussed in the earlier section on biofuels, agricultural systems are typically characterized by a yield (Y) which corresponds to the efficiency of production of some agricultural product per unit area (A). For example, units for yield often bushels per acre, tons per hectare, pounds per square foot, etc. For terrestrial systems, land is a key requirement but it’s really the soil that is critical to the process and often overlooked by those not working within agricultural systems. Without good soil, yield of food products are not sustainable. Soil is a critical part of the ecosystem on land and it is a primary determinant of which food products are grown in which locations.
Improvements in crop productivity is one of the solutions detailed by the WRI food report. Over the years, improvements in crop breeding by selection of better strains of corn (figure 5.3).
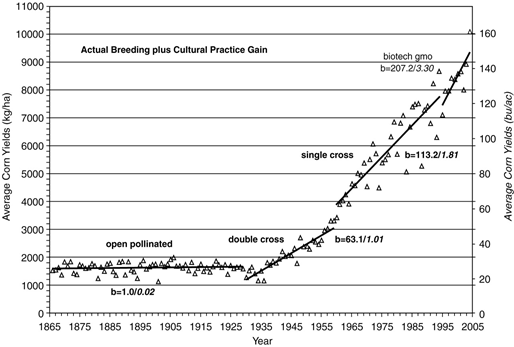
Figure 5.3: Historical increase in Corn Yields since the 1860s. Source: Troyer, A. F. 2006. Adaptedness and Heterosis in Corn and Mule Hybrids. Crop Science, v. 46, n. 2, p. 528-543
The 5 basic requirements for plants are nitrogen, potassium, phosphorus, water and sunlight. Nitrogen is the almost 80% of the composition of air, but due to its strong triple bond, it is not available for plants to use directly from the air. Instead, plants get the nitrogen that they need from the soil, where it has already been converted, or “fixed”, from molecular (N2) to ammonia (NH3) by bacteria and archaea. The soil is the key to a healthy agricultural environmental. While we might take the soil for granted, it is a key natural capital resource. Without high quality soil, agriculture is not possible. Healthy soil is a dynamic living ecosystem which contains a complex mixture of minerals, organic matter, moisture, and a diverse set of living organisms.
Soil organic matter, or soil carbon, is critical to farmers for a number of reasons including sequestering carbon and increasing crop yields. Carbon in the soil supports a number of microorganisms which provide the nutrients needed for plant growth. Increased soil carbon is correlated with higher crop yields. The process is complex, but as microorganisms thrive on organic material, more nitrogen is made available for the plants. Organic material in the soil can also help to hold more moisture in the soil. It is estimated that every 1% increase in soil organic matter allows soil hold 20,000 gallons more water per acre (Source).
Topsoil is generally considered the top 6 – 12 inches of the soil which contains the majority of the organic material and microorganisms needed for plant growth. Since farmers began tilling the land in the US approximately 150 years ago, more than 50 billion metric tons of topsoil have eroded, an erosion rate double what the U.S. Department of Agriculture (USDA) says is sustainable. This erosion is primarily due to poor agricultural practices which lead to due higher erosion by wind and water.
There are a variety of ways to maintain topsoil and increase soil carbon which is considered a method of carbon sequestration. Reduced tillage or no-till agriculture is known to increase soil carbon. Tillage is the preparation of soil by mechanical means such as plowing and overturning of the soil. This process exposes more of the organic material in the soil to the sun, water, and air which results in more oxidation of carbon to carbon dioxide.
Converting natural ecosystems like forests and grasslands to farmland disturbs the fundamental soil structure and releases much of that stored carbon into the atmosphere. This is in addition to the loss of the growing trees and grasses which store carbon in their woody structure as they continue to grow.
A recent Nature Sustainability article estimates that soil carbon represents 25% of the potential of natural climate solutions for sequestration of carbon. The soil has the potential to sequester almost 24 Gt of CO2-equivalent per year, 40% of which is the protection of existing soil carbon and 60% is the rebuilding depleted soil stocks.
Agriculture also depletes the soil of nitrogen since harvesting of the crops removes the nitrogen from the soil at a rate faster than it is regenerated by bacteria in the soil. This becomes a much more significant problem as the scale of farming increases. Nitrogen is one of the limited factors for the growth of plants. Smaller scale farming, which was the predominant method of farming before large factory farms took over in the past 50 years, used natural fertilizers from animal and crop residues.
The Haber-Bosch process, for which Fritz Haber and Carl Bosch won the Nobel Prize, produces ammonia economically from nitrogen and hydrogen under high pressures and moderately high temperatures in the presence of an active catalyst. This synthetic route for ammonia opened up the possibility for cheaper nitrogen-based fertilizers at very large scale. It allows the production of food at much higher scales and alleviated some food insecurity in some locations around the world.
There are serious environmental concerns with the widespread use of synthetic fertilizer. When cheap and available, it is often over used on the land so that much of it (how much) ends up in the air or water. Nitrous oxide, N2O,is a powerful greenhouse gases, 260 times more powerful than CO2. Nitrogen fertilizer speeds up the breakdown of organic matter which leads to lower microbial populations. Nitrogen oxides also create acid rain and make soils more acidic. Nitrogen compounds also run off into rivers, where they promote the growth of algae blooms which lead to ocean “dead zones” as the decaying algae decomposes by depleting the oxygen from water near the sea floor. Some algae also causes health affects for humans due to chemicals in the air and water.
Pesticides are also chemical technologies which kills pests that reduce crops yields. These have become more and more necessary as farming has increased in scale and shifted to monoculture in which only one crop is grown over huge areas. This makes it easy for insects and other pests to thrive in farming environments. Pesticides, however, has a number of issues. The reduce biodiversity which is important for a health ecosystem since most pesticides cannot kills just one or two specific pests, but rather kill most insects or mammals. Most pests also adapt or evolve to pesticides in order to survive. Like antibiotic resistant bacteria and viruses, some insects, bacteria, and fungi are able to develop some resistance to pesticide which just increases the chemical warfare needed to manage the pests. Moreover, most pesticides have health concerns at higher concentrations for humans and other large animals in the ecosystem. Most fruits and vegetables in the supermarket which are not organic have pesticide residue. In some cases, the levels of pesticides are thought to have long-term risks to those eating these foods. Further upstream in the life cycle, workers who harvest or process food products for many hours a day are exposed to even higher concentrations of pesticides. Other pesticides end up in water runoff and affect aquatic ecosystems or end up in rivers, lakes, and other drinking water sources from which they must be removed.
Organic farming limits the use of synthetic pesticides to significantly reduce these chemicals on the food and in the environment. Crop yields, however, are difficult to maintain without synthetic pesticides using other natural chemicals or organisms to deter pests. Lower crops yields and more intensive agricultural practices other than relatively cheap and easily spray application of pesticides leads to higher costs for most organic foods.
5.2.1.1 Food Waste
Food Waste - are we part of the problem? Think about what the produce you left in your refrigerator that you forgot about, or yogurt that you only ate 1/2 the container by the sell by date. Here in the U.S., each of us throws out food from our fridgerators. Food is also wasted at the Grocery Stores (see store grades), and at the farm fields. In contrast, in other parts of the world a larger amount of food goes bad while in storage (through varmints, inadequate refrigeration) prior to getting to the people. Worldwide, over 1/3 of food is wasted. And yet we have peeople across our planet that are hungry when they wake up and hungry when they go to bed.
Grocery Store Food Waste Efforts
Store | Grade |
---|---|
Walmart | B |
Kroger | C |
Food Lion | C |
CostCo | D |
Trader Joes | D |
Whole Foods | D |
Target | D |
Source: NPR
Food waste is a major issue across the life cycle of food production. Food that is produced and not consumed are lost calories and contribute to the food gap, especially in food insecure areas. Estimates suggest that more than 25% of food grown for human consumption is not eaten. This waste of food occurs all across the food chain as shown in Figure 5.4. In developed countries, food waste is higher and dominated in the consumption stage while in developing countries the waste is highest in the food production, handling, and storage categories. In all countries, there are significant opportunities to reduce food waste.
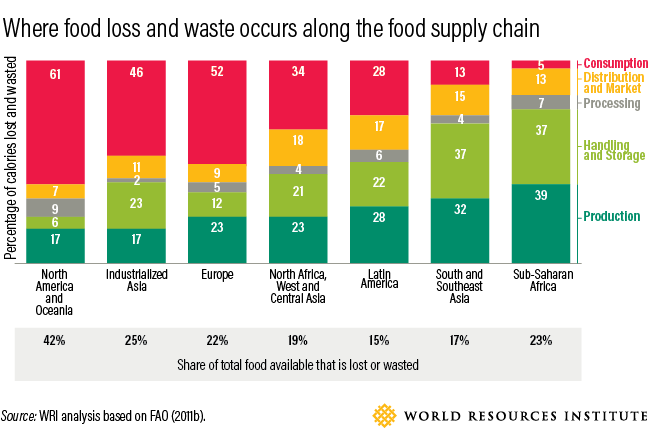
Figure 5.4: Food Loss and Waste in the Food Chain. Source: World Resources Institute (WRI) - https://www.wri.org/insights/how-sustainably-feed-10-billion-people-2050-21-charts
While we can easily see this food waste from our individual meals, there are typically larger food waste amounts in the upstream agricultural or food processing steps. Unless we work in these areas, this waste is largely unseen by the public. Animal farms for poultry, chicken, beef, etc. generate large amounts of animal waste daily which must be managed for large scale operations. Historically, for smaller farms, animal wastes (manure) were used as fertilizer for farm operations in a sustainable manner. However, as farms have become much larger and more concentrated for efficiency over the last 50 years, the amounts of manure and animal wastes are too much for on-farm use and must be collected since runoff can cause environmental damage due to excessive nitrogen and phosphorus loads.
The issue of food waste is exacerbated the disposal of food products that are thrown away at various stages of the food chain and end up in the landfill. This organic material contributes to GHG emissions since food products in the landfill are decomposed anaerobically (in the absence of oxygen) by microbes which produce methane. Separating food waste from the landfill stream is a good solution to this problem, but depends both on individual, commercial, and industrial scale behaviors and processes to avoid the landfill which is by far the easiest (and often least expensive) method of disposal.
5.2.1.2 Food Miles
The concept of food-miles is the distance from the agricultural/food production system to the household table. This is easy for the average citizen to grasp. Simplistically, reducing the amount of food miles seems advantageous for several reasons. You may have even heard that “the produce in the typical American supermarket travels and average 1,500 miles from field to bin” which is a bit shocking. Local food systems can reduce food miles which provides energy and transportation savings. Consumers also benefit from fresher, better-tasting, and more nutritious food, while more food dollars stay within rural communities.
Considering the life cycle makes this analysis more complex. It is not only the distance that the food travels that matters, but also the efficiency of the transportation mode and the time to market. A more detailed life cycle analysis is required for a more thorough analysis.
In a detailed study of food systems (Food-Miles and the Relative Climate Impacts of Food Choices in the United States, Christopher L. Weber, and H. Scott Matthews, Carnegie Mellon University, Environ. Sci. Technol., 2008, 42 (10), 3508-3513). the authors found that US food is transported long distances, on average, with approximately 1640 km (1020 miles) just for delivery and 6760 km (4200 miles) for the full life-cycle supply chain. Despite this fact, the GHG emissions associated with food are dominated by the production phase, contributing 83% of the average U.S. household’s 8.1 ton CO2e per yr footprint for food consumption. Transportation, as a whole represents, only 11/% of life-cycle GHG emissions, and final delivery from producer to retail contributes only 4%. This means that while lower food miles can reduce energy and GHG emissions as well as have other TBL benefits, transportation is not the phase of the system which has the most opportunity for improvement if energy and emissions are the goal. This is shown in the global food emissions plot in Figure 5.5. Land Use and Agricultural Production dominate the life cycle GHG emissions while Transportation is seen to be a much smaller contributor.
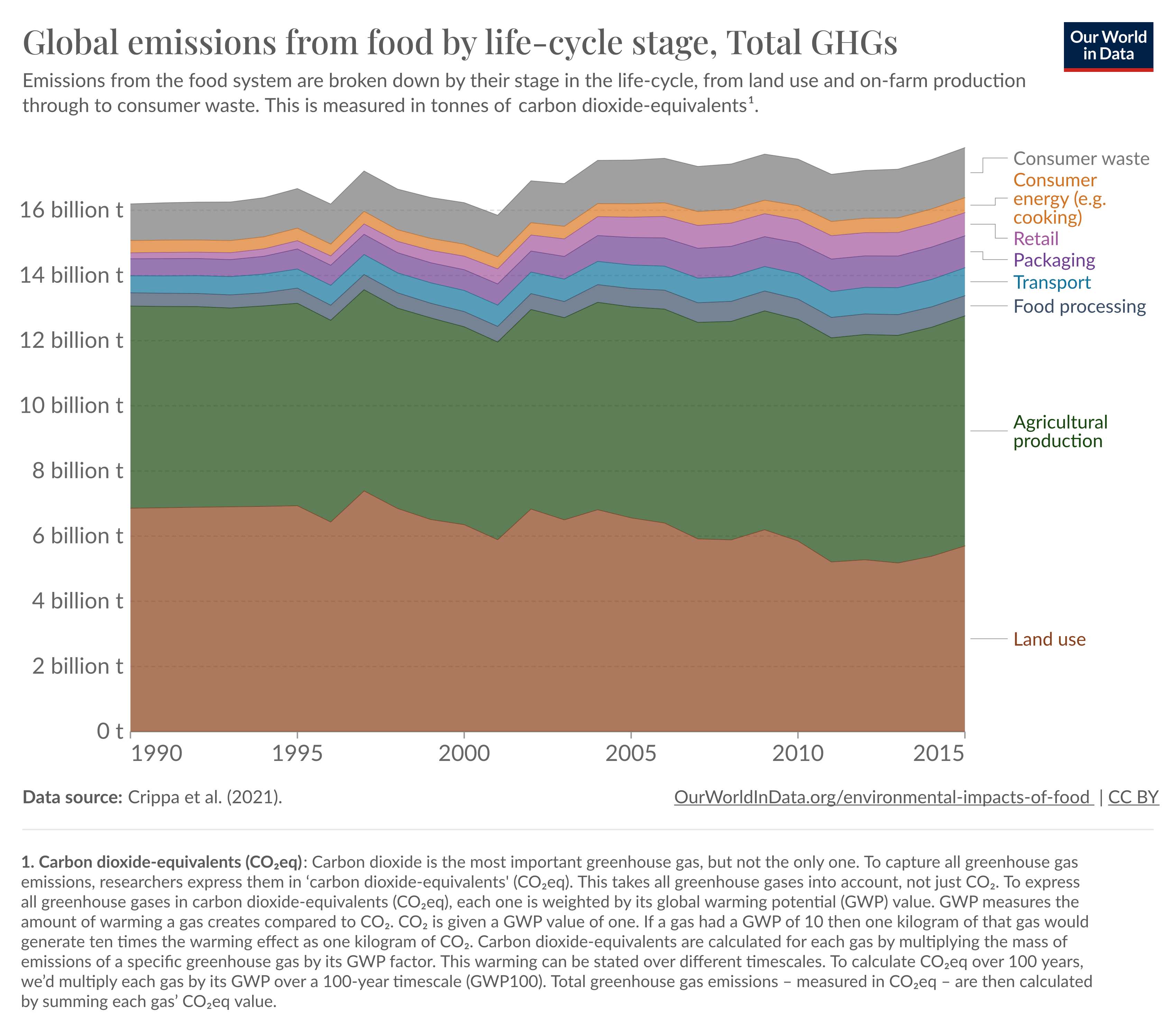
Figure 5.5: Life Cycle GHG Emissions. Source: https://ourworldindata.org/grapher/food-emissions-life-cycle
5.2.2 Solutions
5.2.2.1 Precision Agriculture
Precision agriculture is a general term covering many different uses of technology and engineering to enhance crop yields and efficiency of energy and resources. It uses new technologies to increase crop yields and reduce the levels of traditional inputs needed to grow crops. This increases agricultural efficiency (more output with less input) and therefore also cost. Precision farming tools are based on observations, measurements, analysis, and finally decision-making at the field level. Sometimes this can be done in real-time. Global Positioning Systems (GPS) allow measurements of moisture, fertilizer, and other agricultural parameters as a function of location. GPS devices on tractors, also known as autosteer, allow farmers to plant crops over a given land area in more efficient patterns saving time, fuel, and money (Source). It can plant seeds and apply herbicide/fertilizer with significantly improved precision resulting in fewer overlaps or gaps. For example, if poultry litter or inorganic fertilizer overlaps and gaps were reduced by 20 percent, this would have substantial water quality benefits by reducing the potential for nutrient runoff. Variable rates of fertilizer or herbicides can also be applied based on specific features of the land or crops which vary with location in large fields.
These technologies are currently used primarily by large-scale farms due to costs and complexity of implementing the new technology. However, as costs of new technology come down and are made simpler, it is expected that these technologies will be implemented at smaller farms as well.
Roughly 40% of water use in the US goes to agriculture. Current irrigation methods that use spray or furrows is quite wasteful with significant water lost to evaporation and runoff. Water efficiency for crop irrigation is hard to measure since the water is not physically lost, but rather doesn’t effective provide water in the root zone for plants. It is estimated that sprinklers are only 65-75% efficient. Drip irrigation, on the other hand, is a significant water-saving technology with efficiency of upwards of 90%. From a technical point of view, drip irrigation needs a lower power pump that spray systems since it only needs to provide enough pressure to deliver water drops at some distance from the water source. Drip irrigation is generally limited to smaller agricultural applications due to the need for fixed pipes to be installed and maintained throughout a field in order to efficiently apply water directly at the plant roots.
Drones are another emerging technology agricultural applications which have many applications and opportunities which are not yet fully realized. Implementation is moving slowly, currently, due to regulations and approvals from the Federal Aviation Administration (FAA) to maintain safety. Drones can be operated remotely at a fraction of the cost of planes and satellites used for some agricultural activities and decision-making. Beyond the obvious use of drones to apply pesticides and fertilizers as higher precision than by using aircraft, they will be able to monitor field health from more or less continuous and real time imaging.
Beyond drones, no-flying robots are being developed to do the work traditionally done by hand or large machinery more efficiently. For example, checking for pest test strips in fields typically requires manually monitoring, cleaning, and resetting. Robotic technology allows farmers to track pests from their cellphones in real-time, allowing them to reduce the amount of pesticides sprayed on crops, which saves the farm money and labor.
From a triple bottom line perspective, we must also consider that these new technologies could significantly reduce employment opportunities for many low-income workers in the farm sector.
5.2.2.2 Composting
As we learned in the water module, food production requires water, energy, and people power to grow and distribute. Interventions along the way to “catch” food prior to being tossed requires ingenuity and provides opportunities. For example, an individual may dumpster dive behind a grocery store, or create a mechanism to bring the food to a place for distribution (see https://www.boulderfoodrescue.org). Each of us can compost in our own homes and use it in our yards. When food waste can be separated, composting (Figure 5.6) is one solution to this issue. In composting, food waste and other agricultural products (grass, sawdust, leaves, etc) are mixed and exposed to air. Moisture, and sunlight in outdoor conditions so that they decompose aerobically. This creates some carbon dioxide due to oxidation, but much less methane, and the resulting compost mixture is like the rich carbon-containing humus created in nature due to decomposing organic matter in the soil.
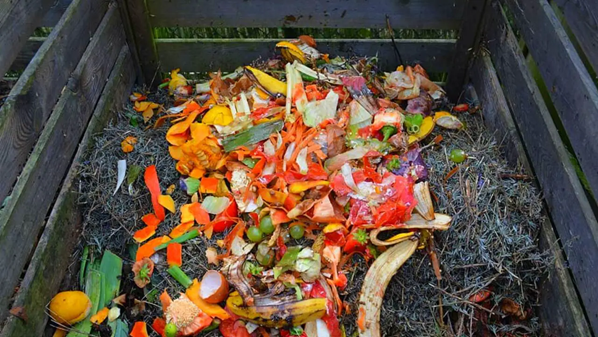
Figure 5.6: Residential Composting Example. Source: https://slickgarden.com/make-compost-at-home-using-kitchen-waste/
For households and individuals, composting requires a bit of outdoor space, which is limiting for many urban and apartment dwellers, as well as a major shift in waste handling. Animal-based products like meat are generally not composted in small system since they have more pungent smells as they decompose and often attract animals. Small composting systems take up just a few square feet and are low cost (Figure 5.7. There is also a small amount of maintenance since the compost piles must be rotated and mixed occasionally to aerate the materials for proper decomposition. Moreover, for optimum decomposition, the compost needs the proper mix of fresh and dry organic material as well as the proper moisture content. When done properly, food and other organic material can turn into finished compost in a month or two which can be used to amend soil in a garden or for landscaping around a house. Few communities in the US offer curbside collection of food waste for composting, so this must be done at an individual level which makes it more difficult to get widespread implementation.
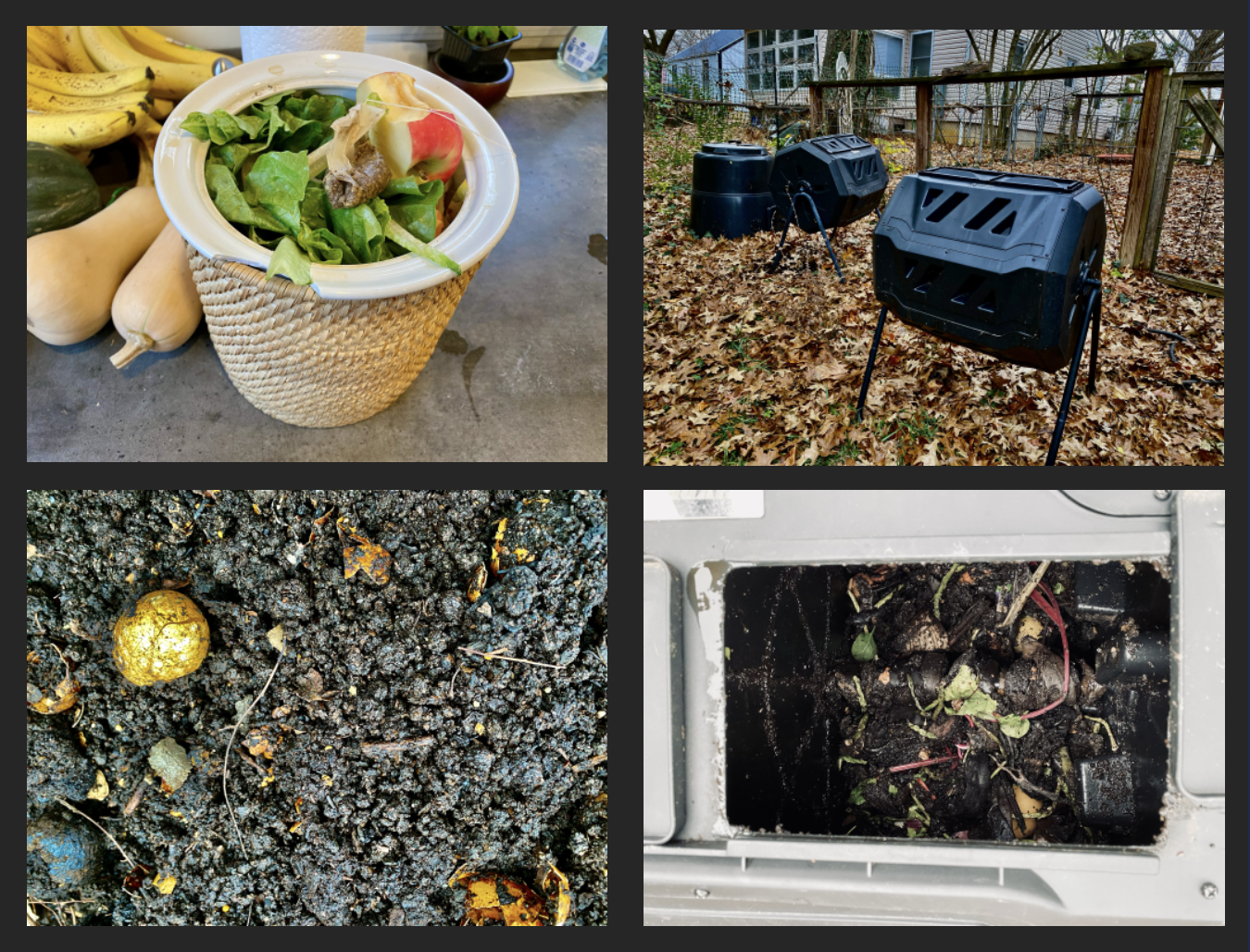
Figure 5.7: Composting from sink-side to final compost. The Scotts have 3-units which are rotated from visible vegatable scraps to the composted product over 3-months. Note the egg shells do nott break down, and sometimes we find avacado skins.
As you can imagine from your experiences in dining halls and restaurants, food waste collection is easier on the kitchen/prep side of the system compared to the waste side. Dining halls often require individual patrons to separate and dispose of food waste in the proper receptacles. With a bit more technology and cost, automated plate and tray system can have the food waste separated by hand centrally rather than depending on individual choices. In restaurants, the added burden of separating the food waste and storing them is often a major barrier to implementation.
On a larger scale, composting follows the same basic methods but gets more labor and equipment intensive to handle big amounts of waste (Figure 5.8). Examples of this would be food waste from a college dining hall, restaurant, or grocery store in which hundreds of pounds or tons of waste are generated in a day or week. Meat and animal products can be composted in these systems given the higher temperatures that are generated to decompose the material and kill unwanted bacteria. In these cases, the logistics are more complicated as the food waste needs to be stored for some period of time until a full load is accumulated and then transported to an off-site facility. At large scale composting facilities, the challenge is managed the large flow of materials. Dump trucks, front end loaders, and bulldozers are required to move the materials to piles. The large compost piles must be aerated using large equipment rather than be hand. Wet food waste can also leach liquids which must be dealt with and the smell of the decomposing material can be an issue depending on the location. These system generate large amounts of finished mulch/compost which can be sold for a profit.
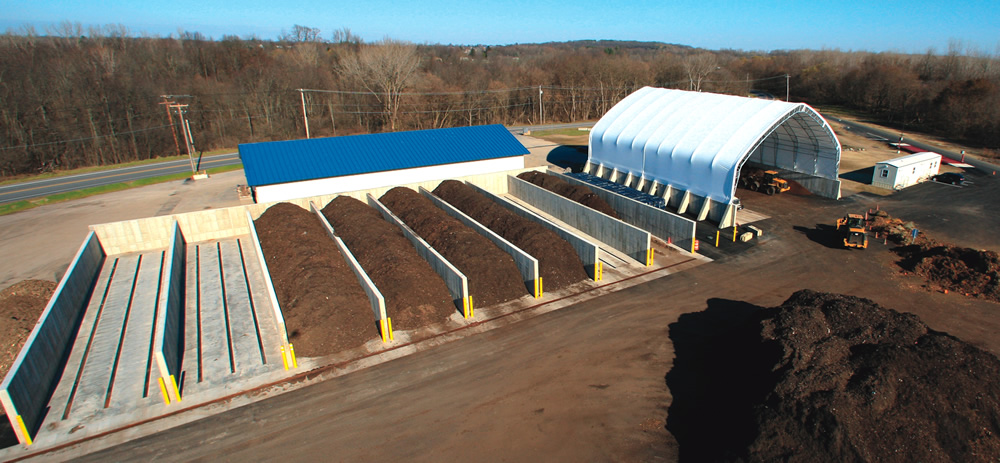
Figure 5.8: Industrial Composting Example. Source: https://www.biocycle.net/smooth-transition-to-food-waste-composting/
5.2.2.3 Modify Diets
Different food groups exhibit a large range in GHG intensity; on average, red meat is around 150% more GHG intensive than chicken or fish. This means that dietary shifts can be a more effective means of lowering an average household’s food-related climate footprint than “buying local.” Shifting less than one day per week’s worth of calories from red meat and dairy products to chicken, fish, eggs, or a vegetable-based diet achieves more GHG reduction than buying all locally-sourced food.”
5.2.2.4 Improve Cattle Feed
We’ve all ready about cows and their flatuence, and the methane emissions directly from cows. In the U.S. alone, there are almost 30million beef cows and over 9 million dairy cows. Researchers here at VT and other land-grants have studied approaches to minimize methane emissions. The basic approach is to make adjustments to the cow’s diet. Source: Drawdown
5.2.2.5 Regenerative Agriculture
The 6 principles of regenerative agriculture as descrinbed by Kiss the Ground.
- Understand Context - the people (community) and the place (weather, habitat)
- Mimimize Disturbance - directly related to Green Engineering, reducing energy and material inputs (water, fertilizer, chemicals)
- Roots - provide carbon to build soil
- Soil Armor - reduce erosion by protecting bare soil
- Integrate Animals - animal grazing can provide services that reduce inputs
- Increase Biodiversity
Glade Road Growing is a local farm located here in Blacksburg. The farm was started by Sally Walker and JP (Jason Pall). If you’ve been out to Rising Silo, you’ve been at Glade Road. They grow vegetables and raise chickens and pigs. They’ve also integrated the local community into their business. Check out their website, and answer the following:
What other benefits does their business provide from a Triple Bottom Line or Donut perspective?
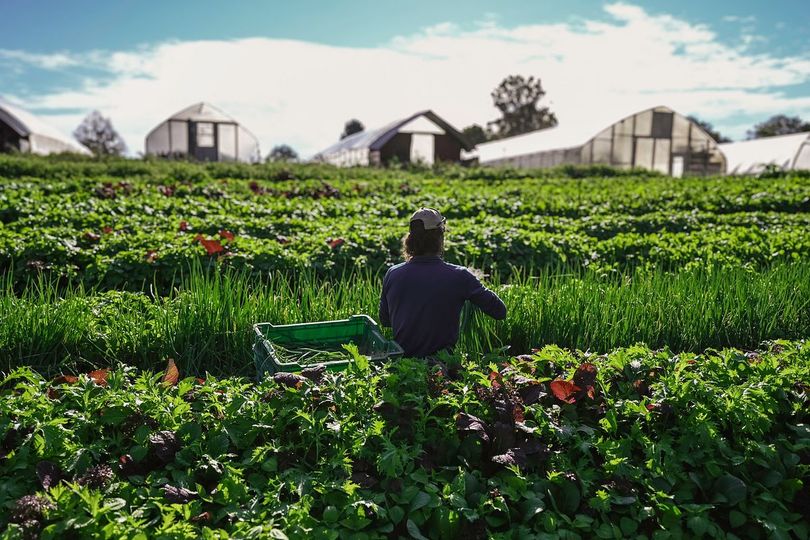